Recognizing the Sines of Infection: Regulation of Retrotransposon Expression and Modulation of Host Cell Processes
Total Page:16
File Type:pdf, Size:1020Kb
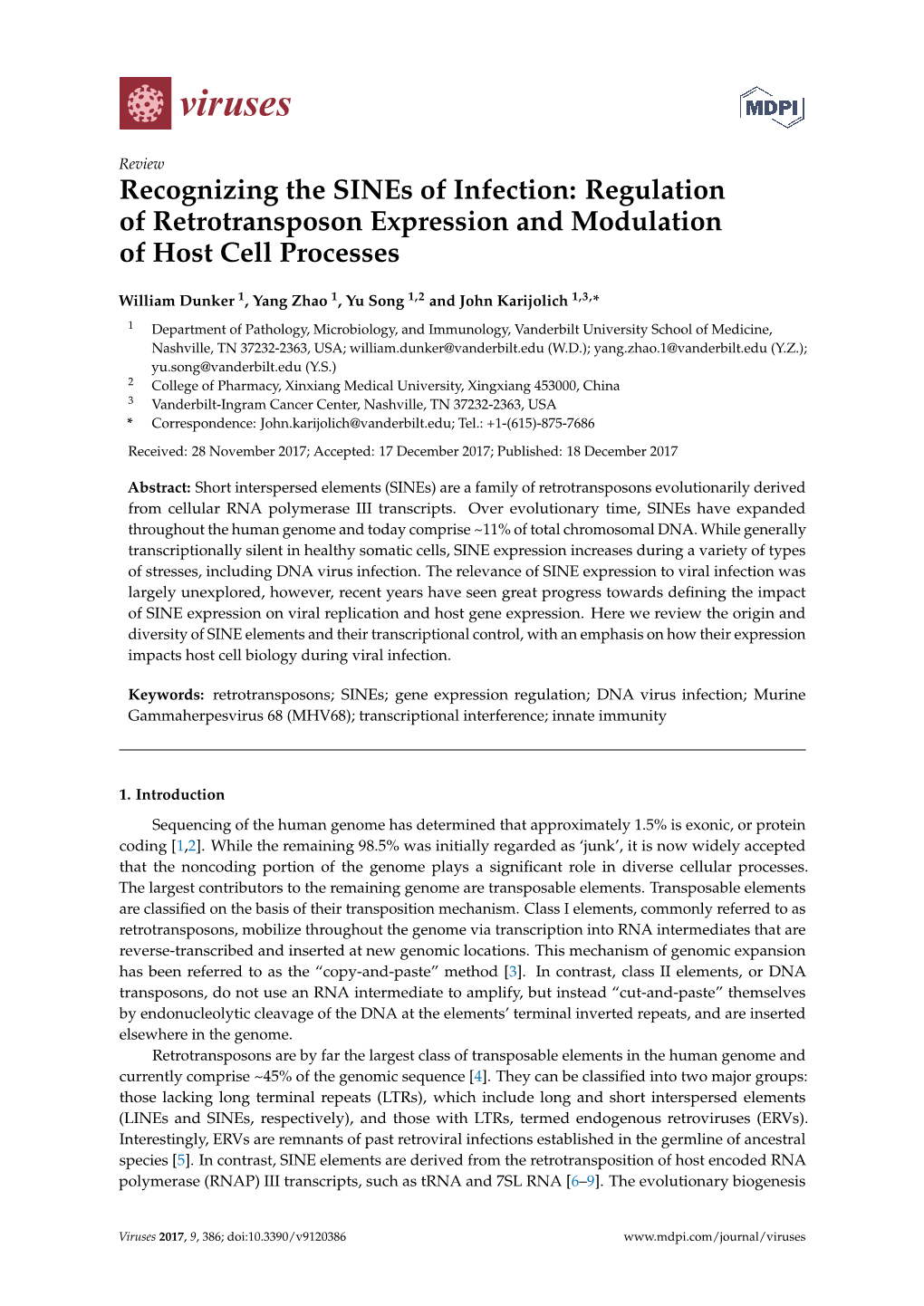
Load more
Recommended publications
-
Autism Multiplex Family with 16P11.2P12.2 Microduplication Syndrome in Monozygotic Twins and Distal 16P11.2 Deletion in Their Brother
European Journal of Human Genetics (2012) 20, 540–546 & 2012 Macmillan Publishers Limited All rights reserved 1018-4813/12 www.nature.com/ejhg ARTICLE Autism multiplex family with 16p11.2p12.2 microduplication syndrome in monozygotic twins and distal 16p11.2 deletion in their brother Anne-Claude Tabet1,2,3,4, Marion Pilorge2,3,4, Richard Delorme5,6,Fre´de´rique Amsellem5,6, Jean-Marc Pinard7, Marion Leboyer6,8,9, Alain Verloes10, Brigitte Benzacken1,11,12 and Catalina Betancur*,2,3,4 The pericentromeric region of chromosome 16p is rich in segmental duplications that predispose to rearrangements through non-allelic homologous recombination. Several recurrent copy number variations have been described recently in chromosome 16p. 16p11.2 rearrangements (29.5–30.1 Mb) are associated with autism, intellectual disability (ID) and other neurodevelopmental disorders. Another recognizable but less common microdeletion syndrome in 16p11.2p12.2 (21.4 to 28.5–30.1 Mb) has been described in six individuals with ID, whereas apparently reciprocal duplications, studied by standard cytogenetic and fluorescence in situ hybridization techniques, have been reported in three patients with autism spectrum disorders. Here, we report a multiplex family with three boys affected with autism, including two monozygotic twins carrying a de novo 16p11.2p12.2 duplication of 8.95 Mb (21.28–30.23 Mb) characterized by single-nucleotide polymorphism array, encompassing both the 16p11.2 and 16p11.2p12.2 regions. The twins exhibited autism, severe ID, and dysmorphic features, including a triangular face, deep-set eyes, large and prominent nasal bridge, and tall, slender build. The eldest brother presented with autism, mild ID, early-onset obesity and normal craniofacial features, and carried a smaller, overlapping 16p11.2 microdeletion of 847 kb (28.40–29.25 Mb), inherited from his apparently healthy father. -
WO 2019/079361 Al 25 April 2019 (25.04.2019) W 1P O PCT
(12) INTERNATIONAL APPLICATION PUBLISHED UNDER THE PATENT COOPERATION TREATY (PCT) (19) World Intellectual Property Organization I International Bureau (10) International Publication Number (43) International Publication Date WO 2019/079361 Al 25 April 2019 (25.04.2019) W 1P O PCT (51) International Patent Classification: CA, CH, CL, CN, CO, CR, CU, CZ, DE, DJ, DK, DM, DO, C12Q 1/68 (2018.01) A61P 31/18 (2006.01) DZ, EC, EE, EG, ES, FI, GB, GD, GE, GH, GM, GT, HN, C12Q 1/70 (2006.01) HR, HU, ID, IL, IN, IR, IS, JO, JP, KE, KG, KH, KN, KP, KR, KW, KZ, LA, LC, LK, LR, LS, LU, LY, MA, MD, ME, (21) International Application Number: MG, MK, MN, MW, MX, MY, MZ, NA, NG, NI, NO, NZ, PCT/US2018/056167 OM, PA, PE, PG, PH, PL, PT, QA, RO, RS, RU, RW, SA, (22) International Filing Date: SC, SD, SE, SG, SK, SL, SM, ST, SV, SY, TH, TJ, TM, TN, 16 October 2018 (16. 10.2018) TR, TT, TZ, UA, UG, US, UZ, VC, VN, ZA, ZM, ZW. (25) Filing Language: English (84) Designated States (unless otherwise indicated, for every kind of regional protection available): ARIPO (BW, GH, (26) Publication Language: English GM, KE, LR, LS, MW, MZ, NA, RW, SD, SL, ST, SZ, TZ, (30) Priority Data: UG, ZM, ZW), Eurasian (AM, AZ, BY, KG, KZ, RU, TJ, 62/573,025 16 October 2017 (16. 10.2017) US TM), European (AL, AT, BE, BG, CH, CY, CZ, DE, DK, EE, ES, FI, FR, GB, GR, HR, HU, ΓΕ , IS, IT, LT, LU, LV, (71) Applicant: MASSACHUSETTS INSTITUTE OF MC, MK, MT, NL, NO, PL, PT, RO, RS, SE, SI, SK, SM, TECHNOLOGY [US/US]; 77 Massachusetts Avenue, TR), OAPI (BF, BJ, CF, CG, CI, CM, GA, GN, GQ, GW, Cambridge, Massachusetts 02139 (US). -
Whole-Genome Expression Analysis of Mammalian-Wide Interspersed
Dna Research, 2017, 24(1), 59–69 doi: 10.1093/dnares/dsw048 Advance Access Publication Date: 17 December 2016 Full Paper Full Paper Whole-genome expression analysis of mammalian-wide interspersed repeat elements in human cell lines Davide Carnevali1, Anastasia Conti1,†, Matteo Pellegrini2 and Giorgio Dieci1,* 1Department of Life Sciences, University of Parma, Parma, Italy, and 2Department of Molecular, Cell, and Developmental Biology, University of California, Los Angeles, CA 90095 723, USA *To whom correspondence should be addressed. Tel. þ39 0521 905649. Fax. þ39 0521 905151. Email: [email protected] †Present address: The San Raffaele Telethon Institute for Gene Therapy (SR-TIGET), Milano, Italy Edited by Dr. Minoru Ko Received 13 August 2016; Accepted 9 October 2016 Abstract With more than 500,000 copies, mammalian-wide interspersed repeats (MIRs), a sub-group of SINEs, represent 2.5% of the human genome and one of the most numerous family of poten- tial targets for the RNA polymerase (Pol) III transcription machinery. Since MIR elements ceased to amplify 130 myr ago, previous studies primarily focused on their genomic impact, while the issue of their expression has not been extensively addressed. We applied a dedicated bioin- formatic pipeline to ENCODE RNA-Seq datasets of seven human cell lines and, for the first time, we were able to define the Pol III-driven MIR transcriptome at single-locus resolution. While the majority of Pol III-transcribed MIR elements are cell-specific, we discovered a small set of ubiquitously transcribed MIRs mapping within Pol II-transcribed genes in antisense orien- tation that could influence the expression of the overlapping gene. -
'Distal 16P12.2 Microdeletion' in a Patient with Autosomal Recessive
Journal of Genetics (2019) 98:56 © Indian Academy of Sciences https://doi.org/10.1007/s12041-019-1107-0 RESEARCH NOTE ‘Distal 16p12.2 microdeletion’ in a patient with autosomal recessive deafness-22 ELISA TASSANO1,∗, PATRIZIA RONCHETTO1, ANNALISA CALCAGNO2, PATRIZIA FIORIO1, GIORGIO GIMELLI1, VALERIA CAPRA3 and MARCELLO SCALA3 1 Laboratorio di Citogenetica, and 2 Department of Pediatrics, and 3 UOC Neurochirurgia, Istituto Giannina Gaslini, 16147 Genova, Italy *For correspondence. E-mail: [email protected] Received 7 January 2019; revised 30 January 2019; accepted 31 January 2019; published online 1 June 2019 Abstract. The 16p12.2 chromosome band contains three large segmental duplications: BP1, BP2 and BP3, providing a substrate for recombination and recurrent chromosomal rearrangements. The ‘16p12.2 microdeletion’ is a recurrent deletion comprised between BP2 and BP3, associated with variable clinical findings. We identified a heterozygous 16p12.2 microdeletion spanning between BP1 and BP2 in a child evaluated for short stature and mild dyslexia. Unexpectedly, the mother carried the same deletion in the homozygous state and suffered from severe hearing loss. Detailed family history revealed consanguinity of the maternal grandparents. The 16p12.2 microdeletion is a rare condition and contains only three genes: METTL9, IGSF6 and OTOA of which the OTOA is considered responsible for DFNB22 hearing loss (MIM: 607039) under its homozygous condition. A number of OTOA mutations have been described, whereas very few cases of a 16p12.2 microdeletion similar to that observed in our family have been reported. In conclusion, we describe a rare ‘distal 16p12.2 microdeletion’ widening the phenotypic spectrum associated with the recurrent 16p12.2 microdeletion and support the causative role of OTOA microdeletion in hearing impairment. -
Clinical Utility Gene Card For: 16P12.2 Microdeletion
European Journal of Human Genetics (2017) 25, doi:10.1038/ejhg.2016.158 & 2017 Macmillan Publishers Limited, part of Springer Nature. All rights reserved 1018-4813/17 www.nature.com/ejhg CLINICAL UTILITY GENE CARD Clinical utility gene card for: 16p12.2 microdeletion Lucilla Pizzo1, Joris Andrieux2, David J Amor3 and Santhosh Girirajan*,1,4 European Journal of Human Genetics (2017) 25, doi:10.1038/ejhg.2016.158; published online 16 November 2016 1. DISEASE CHARACTERISTICS and altered brain activity.5 Limited functional information is available 1.1 Name of the disease (synonyms) on PDZD9, C16orf52 and VWA3A. Even though the basic functionality 16p12.1 microdeletion (hg18/NCBI36). Please note that the updated has been reported for some genes within the region, their exact role release of the human reference genome (hg19/GRCh37) annotates this towards pathogenicity owing to the 16p12.2 deletion is not known, region as 16p12.2. highlighting the need for in-depth molecular studies of these genes. 1.2 OMIM# of the disease 1.6 Analytical methods 136570. The presence of the deletion can be detected by genome-wide or 1.3 Name of the analysed genes or DNA/chromosome segments targeted approaches that determine copy number in the region. Recent Chromosome 16p12.2 (hg19 chr16:g.(?_ 21950000)_(22470000_?)del). studies using genome-wide technologies such as comparative genomic hybridization and SNP microarrays have enabled discovery of this microdeletion, which otherwise would have been missed by lower 1.4 OMIM# of the gene(s) resolution assays such as chromosomal banding techniques. The UQCRC2 (*191329); EEF2K (*606968); CDR2 (*117340); PDZD9 (no accuracy of estimation of the deletion size depends on the type of OMIM entry); C16orf52 (no OMIM entry); VWA3A (no OMIM microarray used (targeted or whole genome) and the density of probes entry); and POLR3E (no OMIM entry). -
Characterizing Genomic Duplication in Autism Spectrum Disorder by Edward James Higginbotham a Thesis Submitted in Conformity
Characterizing Genomic Duplication in Autism Spectrum Disorder by Edward James Higginbotham A thesis submitted in conformity with the requirements for the degree of Master of Science Graduate Department of Molecular Genetics University of Toronto © Copyright by Edward James Higginbotham 2020 i Abstract Characterizing Genomic Duplication in Autism Spectrum Disorder Edward James Higginbotham Master of Science Graduate Department of Molecular Genetics University of Toronto 2020 Duplication, the gain of additional copies of genomic material relative to its ancestral diploid state is yet to achieve full appreciation for its role in human traits and disease. Challenges include accurately genotyping, annotating, and characterizing the properties of duplications, and resolving duplication mechanisms. Whole genome sequencing, in principle, should enable accurate detection of duplications in a single experiment. This thesis makes use of the technology to catalogue disease relevant duplications in the genomes of 2,739 individuals with Autism Spectrum Disorder (ASD) who enrolled in the Autism Speaks MSSNG Project. Fine-mapping the breakpoint junctions of 259 ASD-relevant duplications identified 34 (13.1%) variants with complex genomic structures as well as tandem (193/259, 74.5%) and NAHR- mediated (6/259, 2.3%) duplications. As whole genome sequencing-based studies expand in scale and reach, a continued focus on generating high-quality, standardized duplication data will be prerequisite to addressing their associated biological mechanisms. ii Acknowledgements I thank Dr. Stephen Scherer for his leadership par excellence, his generosity, and for giving me a chance. I am grateful for his investment and the opportunities afforded me, from which I have learned and benefited. I would next thank Drs. -
Dissecting the Regulatory Roles and Cellular Functions of Mammalian Zscan5b and Primate-Specific Paralogs by Younguk Sun Dissert
DISSECTING THE REGULATORY ROLES AND CELLULAR FUNCTIONS OF MAMMALIAN ZSCAN5B AND PRIMATE-SPECIFIC PARALOGS BY YOUNGUK SUN DISSERTATION Submitted in partial fulfillment of the requirements for the degree of Doctor of Philosophy in Cell and Developmental Biology in the Graduate College of the University of Illinois at Urbana-Champaign, 2016 Urbana, Illinois Doctoral Committee: Professor Lisa J. Stubbs, Chair Associate Professor David H. Rivier Associate Professor Craig A. Mizzen Assistant Professor Eric C. Bolton Assistant Professor Rachel Smith-Bolton ABSTRACT Certain subfamilies of zinc finger transcription factors (ZNF-TFs), especially those including KRAB or SCAN protein-interaction domains, have evolved rapidly in mammals due to repeated rounds of gene duplication, deletion, and divergence. The functions of the encoded proteins, and the evolutionary role of their duplication and divergence, are not well understood. I have focused on mammalian ZNF subfamilies that are rooted by relatively unique, conserved members but that have duplicated to generate groups of divergent paralogous transcription factors in primate species. One such family is the ZSCAN5 subfamily, represented by a single, conserved founding member, ZSCAN5B, and clustered paralogs that arose early in primate history, and this clustered subfamily has been the subject of my thesis work. I have shown that three of the primate paralogs, ZSCAN5A, B, and D, are expressed in different tissues and cell types while ZSCAN5C is not detectably expressed in most tissues. Using chromatin immunoprecipitation (ChIP-seq) in human cell lines and mouse placenta tissue, I have identified mammalian ZSCAN5B as a protein that binds with high specificity to tRNA genes (tDNA) and other polymerase III (Pol III) transcripts including several types of transposable elements (TEs). -
MAFB Determines Human Macrophage Anti-Inflammatory
MAFB Determines Human Macrophage Anti-Inflammatory Polarization: Relevance for the Pathogenic Mechanisms Operating in Multicentric Carpotarsal Osteolysis This information is current as of October 4, 2021. Víctor D. Cuevas, Laura Anta, Rafael Samaniego, Emmanuel Orta-Zavalza, Juan Vladimir de la Rosa, Geneviève Baujat, Ángeles Domínguez-Soto, Paloma Sánchez-Mateos, María M. Escribese, Antonio Castrillo, Valérie Cormier-Daire, Miguel A. Vega and Ángel L. Corbí Downloaded from J Immunol 2017; 198:2070-2081; Prepublished online 16 January 2017; doi: 10.4049/jimmunol.1601667 http://www.jimmunol.org/content/198/5/2070 http://www.jimmunol.org/ Supplementary http://www.jimmunol.org/content/suppl/2017/01/15/jimmunol.160166 Material 7.DCSupplemental References This article cites 69 articles, 22 of which you can access for free at: http://www.jimmunol.org/content/198/5/2070.full#ref-list-1 by guest on October 4, 2021 Why The JI? Submit online. • Rapid Reviews! 30 days* from submission to initial decision • No Triage! Every submission reviewed by practicing scientists • Fast Publication! 4 weeks from acceptance to publication *average Subscription Information about subscribing to The Journal of Immunology is online at: http://jimmunol.org/subscription Permissions Submit copyright permission requests at: http://www.aai.org/About/Publications/JI/copyright.html Email Alerts Receive free email-alerts when new articles cite this article. Sign up at: http://jimmunol.org/alerts The Journal of Immunology is published twice each month by The American Association of Immunologists, Inc., 1451 Rockville Pike, Suite 650, Rockville, MD 20852 Copyright © 2017 by The American Association of Immunologists, Inc. All rights reserved. Print ISSN: 0022-1767 Online ISSN: 1550-6606. -
Content Based Search in Gene Expression Databases and a Meta-Analysis of Host Responses to Infection
Content Based Search in Gene Expression Databases and a Meta-analysis of Host Responses to Infection A Thesis Submitted to the Faculty of Drexel University by Francis X. Bell in partial fulfillment of the requirements for the degree of Doctor of Philosophy November 2015 c Copyright 2015 Francis X. Bell. All Rights Reserved. ii Acknowledgments I would like to acknowledge and thank my advisor, Dr. Ahmet Sacan. Without his advice, support, and patience I would not have been able to accomplish all that I have. I would also like to thank my committee members and the Biomed Faculty that have guided me. I would like to give a special thanks for the members of the bioinformatics lab, in particular the members of the Sacan lab: Rehman Qureshi, Daisy Heng Yang, April Chunyu Zhao, and Yiqian Zhou. Thank you for creating a pleasant and friendly environment in the lab. I give the members of my family my sincerest gratitude for all that they have done for me. I cannot begin to repay my parents for their sacrifices. I am eternally grateful for everything they have done. The support of my sisters and their encouragement gave me the strength to persevere to the end. iii Table of Contents LIST OF TABLES.......................................................................... vii LIST OF FIGURES ........................................................................ xiv ABSTRACT ................................................................................ xvii 1. A BRIEF INTRODUCTION TO GENE EXPRESSION............................. 1 1.1 Central Dogma of Molecular Biology........................................... 1 1.1.1 Basic Transfers .......................................................... 1 1.1.2 Uncommon Transfers ................................................... 3 1.2 Gene Expression ................................................................. 4 1.2.1 Estimating Gene Expression ............................................ 4 1.2.2 DNA Microarrays ...................................................... -
Transcriptional Interference by RNA Polymerase III Affects Expression of the Polr3e Gene
Downloaded from genesdev.cshlp.org on September 27, 2021 - Published by Cold Spring Harbor Laboratory Press Transcriptional interference by RNA polymerase III affects expression of the Polr3e gene Meghdad Yeganeh,1 Viviane Praz,1,2 Pascal Cousin,1 and Nouria Hernandez1 1Center for Integrative Genomics, Faculty of Biology and Medicine, University of Lausanne, 1015 Lausanne, Switzerland; 2Swiss Institute of Bioinformatics, 1015 Lausanne, Switzerland Overlapping gene arrangements can potentially contribute to gene expression regulation. A mammalian inter- spersed repeat (MIR) nested in antisense orientation within the first intron of the Polr3e gene, encoding an RNA polymerase III (Pol III) subunit, is conserved in mammals and highly occupied by Pol III. Using a fluorescence assay, CRISPR/Cas9-mediated deletion of the MIR in mouse embryonic stem cells, and chromatin immunoprecipitation assays, we show that the MIR affects Polr3e expression through transcriptional interference. Our study reveals a mechanism by which a Pol II gene can be regulated at the transcription elongation level by transcription of an embedded antisense Pol III gene. [Keywords: RNA polymerase; gene expression regulation; Polr3e gene; antisense transcription; transcriptional interference; SINE] Supplemental material is available for this article. Received November 15, 2016; revised version accepted February 15, 2017. In eukaryotes, RNA polymerase II (Pol II) is responsible for DSIF follows a similar pattern, whereas NELF is typically transcription of all of the mRNA-encoding genes as well found only at the promoter-proximal pause region (Zhou as most genes encoding small nuclear RNA (snRNA) et al. 2012). and microRNAs. Pol II-dependent transcription occurs Genes often lie in overlapping arrangements on either in several steps, each of which can be subjected to regula- the same strand or opposite strands. -
Transcriptional Interference by RNA Polymerase III Affects Expression of the Polr3e Gene
Downloaded from genesdev.cshlp.org on April 6, 2017 - Published by Cold Spring Harbor Laboratory Press Transcriptional interference by RNA polymerase III affects expression of the Polr3e gene Meghdad Yeganeh,1 Viviane Praz,1,2 Pascal Cousin,1 and Nouria Hernandez1 1Center for Integrative Genomics, Faculty of Biology and Medicine, University of Lausanne, 1015 Lausanne, Switzerland; 2Swiss Institute of Bioinformatics, 1015 Lausanne, Switzerland Overlapping gene arrangements can potentially contribute to gene expression regulation. A mammalian inter- spersed repeat (MIR) nested in antisense orientation within the first intron of the Polr3e gene, encoding an RNA polymerase III (Pol III) subunit, is conserved in mammals and highly occupied by Pol III. Using a fluorescence assay, CRISPR/Cas9-mediated deletion of the MIR in mouse embryonic stem cells, and chromatin immunoprecipitation assays, we show that the MIR affects Polr3e expression through transcriptional interference. Our study reveals a mechanism by which a Pol II gene can be regulated at the transcription elongation level by transcription of an embedded antisense Pol III gene. [Keywords: RNA polymerase; gene expression regulation; Polr3e gene; antisense transcription; transcriptional interference; SINE] Supplemental material is available for this article. Received November 15, 2016; revised version accepted February 15, 2017. In eukaryotes, RNA polymerase II (Pol II) is responsible for DSIF follows a similar pattern, whereas NELF is typically transcription of all of the mRNA-encoding genes as well found only at the promoter-proximal pause region (Zhou as most genes encoding small nuclear RNA (snRNA) et al. 2012). and microRNAs. Pol II-dependent transcription occurs Genes often lie in overlapping arrangements on either in several steps, each of which can be subjected to regula- the same strand or opposite strands. -
Clinical Efficacy and Immune Regulation with Peanut Oral
Clinical efficacy and immune regulation with peanut oral immunotherapy Stacie M. Jones, MD,a Laurent Pons, PhD,b Joseph L. Roberts, MD, PhD,b Amy M. Scurlock, MD,a Tamara T. Perry, MD,a Mike Kulis, PhD,b Wayne G. Shreffler, MD, PhD,c Pamela Steele, CPNP,b Karen A. Henry, RN,a Margaret Adair, MD,b James M. Francis, PhD,d Stephen Durham, MD,d Brian P. Vickery, MD,b Xiaoping Zhong, MD, PhD,b and A. Wesley Burks, MDb Little Rock, Ark, Durham, NC, New York, NY, and London, United Kingdom Background: Oral immunotherapy (OIT) has been thought to noted during OIT resolved spontaneously or with induce clinical desensitization to allergenic foods, but trials antihistamines. By 6 months, titrated skin prick tests and coupling the clinical response and immunologic effects of peanut activation of basophils significantly declined. Peanut-specific OIT have not been reported. IgE decreased by 12 to 18 months, whereas IgG4 increased Objective: The study objective was to investigate the clinical significantly. Serum factors inhibited IgE–peanut complex efficacy and immunologic changes associated with OIT. formation in an IgE-facilitated allergen binding assay. Secretion Methods: Children with peanut allergy underwent an OIT of IL-10, IL-5, IFN-g, and TNF-a from PBMCs increased over protocol including initial day escalation, buildup, and a period of 6 to 12 months. Peanut-specific forkhead box protein maintenance phases, and then oral food challenge. Clinical 3 T cells increased until 12 months and decreased thereafter. In response and immunologic changes were evaluated. addition, T-cell microarrays showed downregulation of genes in Results: Of 29 subjects who completed the protocol, 27 ingested apoptotic pathways.