MATH 4030 Differential Geometry Lecture Notes Part 2 After Studying
Total Page:16
File Type:pdf, Size:1020Kb
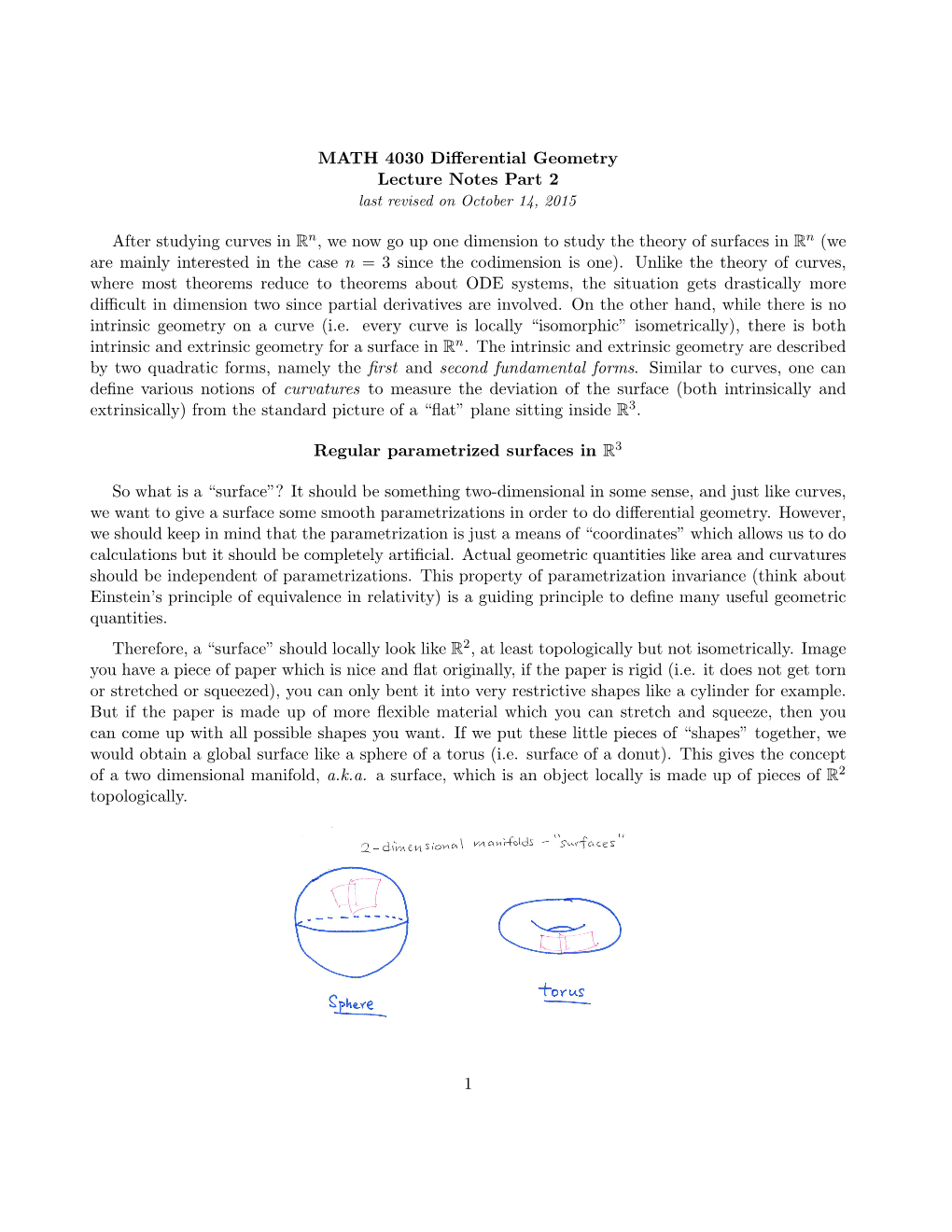
Load more
Recommended publications
-
Toponogov.V.A.Differential.Geometry
Victor Andreevich Toponogov with the editorial assistance of Vladimir Y. Rovenski Differential Geometry of Curves and Surfaces A Concise Guide Birkhauser¨ Boston • Basel • Berlin Victor A. Toponogov (deceased) With the editorial assistance of: Department of Analysis and Geometry Vladimir Y. Rovenski Sobolev Institute of Mathematics Department of Mathematics Siberian Branch of the Russian Academy University of Haifa of Sciences Haifa, Israel Novosibirsk-90, 630090 Russia Cover design by Alex Gerasev. AMS Subject Classification: 53-01, 53Axx, 53A04, 53A05, 53A55, 53B20, 53B21, 53C20, 53C21 Library of Congress Control Number: 2005048111 ISBN-10 0-8176-4384-2 eISBN 0-8176-4402-4 ISBN-13 978-0-8176-4384-3 Printed on acid-free paper. c 2006 Birkhauser¨ Boston All rights reserved. This work may not be translated or copied in whole or in part without the writ- ten permission of the publisher (Birkhauser¨ Boston, c/o Springer Science+Business Media Inc., 233 Spring Street, New York, NY 10013, USA) and the author, except for brief excerpts in connection with reviews or scholarly analysis. Use in connection with any form of information storage and re- trieval, electronic adaptation, computer software, or by similar or dissimilar methodology now known or hereafter developed is forbidden. The use in this publication of trade names, trademarks, service marks and similar terms, even if they are not identified as such, is not to be taken as an expression of opinion as to whether or not they are subject to proprietary rights. Printed in the United States of America. (TXQ/EB) 987654321 www.birkhauser.com Contents Preface ....................................................... vii About the Author ............................................. -
Riemannian Submanifolds: a Survey
RIEMANNIAN SUBMANIFOLDS: A SURVEY BANG-YEN CHEN Contents Chapter 1. Introduction .............................. ...................6 Chapter 2. Nash’s embedding theorem and some related results .........9 2.1. Cartan-Janet’s theorem .......................... ...............10 2.2. Nash’s embedding theorem ......................... .............11 2.3. Isometric immersions with the smallest possible codimension . 8 2.4. Isometric immersions with prescribed Gaussian or Gauss-Kronecker curvature .......................................... ..................12 2.5. Isometric immersions with prescribed mean curvature. ...........13 Chapter 3. Fundamental theorems, basic notions and results ...........14 3.1. Fundamental equations ........................... ..............14 3.2. Fundamental theorems ............................ ..............15 3.3. Basic notions ................................... ................16 3.4. A general inequality ............................. ...............17 3.5. Product immersions .............................. .............. 19 3.6. A relationship between k-Ricci tensor and shape operator . 20 3.7. Completeness of curvature surfaces . ..............22 Chapter 4. Rigidity and reduction theorems . ..............24 4.1. Rigidity ....................................... .................24 4.2. A reduction theorem .............................. ..............25 Chapter 5. Minimal submanifolds ....................... ...............26 arXiv:1307.1875v1 [math.DG] 7 Jul 2013 5.1. First and second variational formulas -
Basics of the Differential Geometry of Surfaces
Chapter 20 Basics of the Differential Geometry of Surfaces 20.1 Introduction The purpose of this chapter is to introduce the reader to some elementary concepts of the differential geometry of surfaces. Our goal is rather modest: We simply want to introduce the concepts needed to understand the notion of Gaussian curvature, mean curvature, principal curvatures, and geodesic lines. Almost all of the material presented in this chapter is based on lectures given by Eugenio Calabi in an upper undergraduate differential geometry course offered in the fall of 1994. Most of the topics covered in this course have been included, except a presentation of the global Gauss–Bonnet–Hopf theorem, some material on special coordinate systems, and Hilbert’s theorem on surfaces of constant negative curvature. What is a surface? A precise answer cannot really be given without introducing the concept of a manifold. An informal answer is to say that a surface is a set of points in R3 such that for every point p on the surface there is a small (perhaps very small) neighborhood U of p that is continuously deformable into a little flat open disk. Thus, a surface should really have some topology. Also,locally,unlessthe point p is “singular,” the surface looks like a plane. Properties of surfaces can be classified into local properties and global prop- erties.Intheolderliterature,thestudyoflocalpropertieswascalled geometry in the small,andthestudyofglobalpropertieswascalledgeometry in the large.Lo- cal properties are the properties that hold in a small neighborhood of a point on a surface. Curvature is a local property. Local properties canbestudiedmoreconve- niently by assuming that the surface is parametrized locally. -
AN INTRODUCTION to the CURVATURE of SURFACES by PHILIP ANTHONY BARILE a Thesis Submitted to the Graduate School-Camden Rutgers
AN INTRODUCTION TO THE CURVATURE OF SURFACES By PHILIP ANTHONY BARILE A thesis submitted to the Graduate School-Camden Rutgers, The State University Of New Jersey in partial fulfillment of the requirements for the degree of Master of Science Graduate Program in Mathematics written under the direction of Haydee Herrera and approved by Camden, NJ January 2009 ABSTRACT OF THE THESIS An Introduction to the Curvature of Surfaces by PHILIP ANTHONY BARILE Thesis Director: Haydee Herrera Curvature is fundamental to the study of differential geometry. It describes different geometrical and topological properties of a surface in R3. Two types of curvature are discussed in this paper: intrinsic and extrinsic. Numerous examples are given which motivate definitions, properties and theorems concerning curvature. ii 1 1 Introduction For surfaces in R3, there are several different ways to measure curvature. Some curvature, like normal curvature, has the property such that it depends on how we embed the surface in R3. Normal curvature is extrinsic; that is, it could not be measured by being on the surface. On the other hand, another measurement of curvature, namely Gauss curvature, does not depend on how we embed the surface in R3. Gauss curvature is intrinsic; that is, it can be measured from on the surface. In order to engage in a discussion about curvature of surfaces, we must introduce some important concepts such as regular surfaces, the tangent plane, the first and second fundamental form, and the Gauss Map. Sections 2,3 and 4 introduce these preliminaries, however, their importance should not be understated as they lay the groundwork for more subtle and advanced topics in differential geometry. -
Convexity, Rigidity, and Reduction of Codimension of Isometric
CONVEXITY, RIGIDITY, AND REDUCTION OF CODIMENSION OF ISOMETRIC IMMERSIONS INTO SPACE FORMS RONALDO F. DE LIMA AND RUBENS L. DE ANDRADE To Elon Lima, in memoriam, and Manfredo do Carmo, on his 90th birthday Abstract. We consider isometric immersions of complete connected Riemann- ian manifolds into space forms of nonzero constant curvature. We prove that if such an immersion is compact and has semi-definite second fundamental form, then it is an embedding with codimension one, its image bounds a convex set, and it is rigid. This result generalizes previous ones by M. do Carmo and E. Lima, as well as by M. do Carmo and F. Warner. It also settles affirma- tively a conjecture by do Carmo and Warner. We establish a similar result for complete isometric immersions satisfying a stronger condition on the second fundamental form. We extend to the context of isometric immersions in space forms a classical theorem for Euclidean hypersurfaces due to Hadamard. In this same context, we prove an existence theorem for hypersurfaces with pre- scribed boundary and vanishing Gauss-Kronecker curvature. Finally, we show that isometric immersions into space forms which are regular outside the set of totally geodesic points admit a reduction of codimension to one. 1. Introduction Convexity and rigidity are among the most essential concepts in the theory of submanifolds. In his work, R. Sacksteder established two fundamental results in- volving these concepts. Combined, they state that for M n a non-flat complete connected Riemannian manifold with nonnegative sectional curvatures, any iso- metric immersion f : M n Rn+1 is, in fact, an embedding and has f(M) as the boundary of a convex set. -
Shapewright: Finite Element Based Free-Form Shape Design
ShapeWright: Finite Element Based Free-Form Shape Design by George Celniker S.M., Mechanical Engineering Massachusetts Institute of Technology January, 1981 B.S., Mechanical Engineering Massachusetts Institute of Technology May, 1979 Submitted to the Department of Mechanical Engineering in Partial Fulfillment of the Requirements for the Degree of Doctor of Philosophy in Mechanical Engineering at the Massachusetts Institute of Technology September, 1990 © Massachusetts Institute of Technology, 1990, All rights reserved. Signature of Author . ... , , , Department of Mechariical Engineering August 3, 1990 Certified by Professor David C. Gossard Thesis Supervisor Accepted by Ain A. Sonin Chairman, Department Committee MASSACHUITTS INSTITOiI OF TECHIvn .:.. NOV 0 8 1990 LIBRARIEb ShapeWright: Finite Element Based Free-Form Shape Design by George Celniker Submitted to the Department of Mechanical Engineering on September, 1990 inpartial fulfillment of the requirements for the degree of Doctor of Philosophy inMechanical Engineering. Abstract The mathematical foundations and an implementation of a new free-form shape design paradigm are developed. The finite element method is applied to generate shapes that minimize a surface energy function subject to user-specified geometric constraints while responding to user-specified forces. Because of the energy minimization these surfaces opportunistically seek globally fair shapes. It is proposed that shape fairness is a global and not a local property. Very fair shapes with C1 continuity are designed. Two finite elements, a curve segment and a triangular surface element, are developed and used as the geometric primitives of the approach. The curve segments yield piecewise C1 Hermite cubic polynomials. The surface element edges have cubic variations in position and parabolic variations in surface normal and can be combined to yield piecewise C1 surfaces. -
Fundamental Forms of Surfaces and the Gauss-Bonnet Theorem
FUNDAMENTAL FORMS OF SURFACES AND THE GAUSS-BONNET THEOREM HUNTER S. CHASE Abstract. We define the first and second fundamental forms of surfaces, ex- ploring their properties as they relate to measuring arc lengths and areas, identifying isometric surfaces, and finding extrema. These forms can be used to define the Gaussian curvature, which is, unlike the first and second fun- damental forms, independent of the parametrization of the surface. Gauss's Egregious Theorem reveals more about the Gaussian curvature, that it de- pends only on the first fundamental form and thus identifies isometries be- tween surfaces. Finally, we present the Gauss-Bonnet Theorem, a remarkable theorem that, in its global form, relates the Gaussian curvature of the surface, a geometric property, to the Euler characteristic of the surface, a topological property. Contents 1. Surfaces and the First Fundamental Form 1 2. The Second Fundamental Form 5 3. Curvature 7 4. The Gauss-Bonnet Theorem 8 Acknowledgments 12 References 12 1. Surfaces and the First Fundamental Form We begin our study by examining two properties of surfaces in R3, called the first and second fundamental forms. First, however, we must understand what we are dealing with when we talk about a surface. Definition 1.1. A smooth surface in R3 is a subset X ⊂ R3 such that each point has a neighborhood U ⊂ X and a map r : V ! R3 from an open set V ⊂ R2 such that • r : V ! U is a homeomorphism. This means that r is a bijection that continously maps V into U, and that the inverse function r−1 exists and is continuous. -
Geometry of Surfaces 1 4.1
M435: INTRODUCTION TO DIFFERENTIAL GEOMETRY MARK POWELL Contents 4. Geometry of Surfaces 1 4.1. Smooth patches 1 4.2. Tangent spaces 3 4.3. Vector fields and covariant derivatives 4 4.4. The normal vector and the Gauss map 7 4.5. Change of parametrisation 7 4.6. Linear Algebra review 14 4.7. First Fundamental Form 14 4.8. Angles 16 4.9. Areas 17 4.10. Effect of change of coordinates on first fundamental form 17 4.11. Isometries and local isometries 18 References 21 4. Geometry of Surfaces 4.1. Smooth patches. Definition 4.1. A smooth patch of a surface in R3 consists of an open subset U ⊆ R2 and a smooth map r: U ! R3 which is a homeomorphism onto its image r(U), and which has injective derivative. The image r(U) is a smooth surface in R3. Recall that the injective derivative condition can be checked by computing ru ^ rv =6 0 everywhere. A patch gives us coordinates (u; v) on our surface. The curves α(t) = r(t; v) and β(t) = r(u; t), for fixed u; v, are the coordinate lines on our surface. The lines of constant longitude and latitude on the surface of the earth are examples. Smooth means that all derivatives exist. In practice, derivatives up to third or fourth order at most are usually sufficient. Example 4.2. The requirement that r be injective means that we sometimes have to restrict our domain, and we may not cover the whole of some compact surface with one patch. -
The Second Fundamental Form. Geodesics. the Curvature Tensor
Differential Geometry Lia Vas The Second Fundamental Form. Geodesics. The Curvature Tensor. The Fundamental Theorem of Surfaces. Manifolds The Second Fundamental Form and the Christoffel symbols. Consider a surface x = @x @x x(u; v): Following the reasoning that x1 and x2 denote the derivatives @u and @v respectively, we denote the second derivatives @2x @2x @2x @2x @u2 by x11; @v@u by x12; @u@v by x21; and @v2 by x22: The terms xij; i; j = 1; 2 can be represented as a linear combination of tangential and normal component. Each of the vectors xij can be repre- sented as a combination of the tangent component (which itself is a combination of vectors x1 and x2) and the normal component (which is a multi- 1 2 ple of the unit normal vector n). Let Γij and Γij denote the coefficients of the tangent component and Lij denote the coefficient with n of vector xij: Thus, 1 2 P k xij = Γijx1 + Γijx2 + Lijn = k Γijxk + Lijn: The formula above is called the Gauss formula. k The coefficients Γij where i; j; k = 1; 2 are called Christoffel symbols and the coefficients Lij; i; j = 1; 2 are called the coefficients of the second fundamental form. Einstein notation and tensors. The term \Einstein notation" refers to the certain summation convention that appears often in differential geometry and its many applications. Consider a formula can be written in terms of a sum over an index that appears in subscript of one and superscript of P k the other variable. For example, xij = k Γijxk + Lijn: In cases like this the summation symbol is omitted. -
Differential Geometry Curves – Surfaces – Manifolds Third Edition
STUDENT MATHEMATICAL LIBRARY Volume 77 Differential Geometry Curves – Surfaces – Manifolds Third Edition Wolfgang Kühnel Differential Geometry Curves–Surfaces– Manifolds http://dx.doi.org/10.1090/stml/077 STUDENT MATHEMATICAL LIBRARY Volume 77 Differential Geometry Curves–Surfaces– Manifolds Third Edition Wolfgang Kühnel Translated by Bruce Hunt American Mathematical Society Providence, Rhode Island Editorial Board Satyan L. Devadoss John Stillwell (Chair) Erica Flapan Serge Tabachnikov Translation from German language edition: Differentialgeometrie by Wolfgang K¨uhnel, c 2013 Springer Vieweg | Springer Fachmedien Wies- baden GmbH JAHR (formerly Vieweg+Teubner). Springer Fachmedien is part of Springer Science+Business Media. All rights reserved. Translated by Bruce Hunt, with corrections and additions by the author. Front and back cover image by Mario B. Schulz. 2010 Mathematics Subject Classification. Primary 53-01. For additional information and updates on this book, visit www.ams.org/bookpages/stml-77 Page 403 constitutes an extension of this copyright page. Library of Congress Cataloging-in-Publication Data K¨uhnel, Wolfgang, 1950– [Differentialgeometrie. English] Differential geometry : curves, surfaces, manifolds / Wolfgang K¨uhnel ; trans- lated by Bruce Hunt.— Third edition. pages cm. — (Student mathematical library ; volume 77) Includes bibliographical references and index. ISBN 978-1-4704-2320-9 (alk. paper) 1. Geometry, Differential. 2. Curves. 3. Surfaces. 4. Manifolds (Mathematics) I. Hunt, Bruce, 1958– II. Title. QA641.K9613 2015 516.36—dc23 2015018451 c 2015 by the American Mathematical Society. All rights reserved. The American Mathematical Society retains all rights except those granted to the United States Government. Printed in the United States of America. ∞ The paper used in this book is acid-free and falls within the guidelines established to ensure permanence and durability. -
Differential Geometry
18.950 (Differential Geometry) Lecture Notes Evan Chen Fall 2015 This is MIT's undergraduate 18.950, instructed by Xin Zhou. The formal name for this class is “Differential Geometry". The permanent URL for this document is http://web.evanchen.cc/ coursework.html, along with all my other course notes. Contents 1 September 10, 20153 1.1 Curves...................................... 3 1.2 Surfaces..................................... 3 1.3 Parametrized Curves.............................. 4 2 September 15, 20155 2.1 Regular curves................................. 5 2.2 Arc Length Parametrization.......................... 6 2.3 Change of Orientation............................. 7 3 2.4 Vector Product in R ............................. 7 2.5 OH GOD NO.................................. 7 2.6 Concrete examples............................... 8 3 September 17, 20159 3.1 Always Cauchy-Schwarz............................ 9 3.2 Curvature.................................... 9 3.3 Torsion ..................................... 9 3.4 Frenet formulas................................. 10 3.5 Convenient Formulas.............................. 11 4 September 22, 2015 12 4.1 Signed Curvature................................ 12 4.2 Evolute of a Curve............................... 12 4.3 Isoperimetric Inequality............................ 13 5 September 24, 2015 15 5.1 Regular Surfaces................................ 15 5.2 Special Cases of Manifolds .......................... 15 6 September 29, 2015 and October 1, 2015 17 6.1 Local Parametrizations ........................... -
Submanifold Geometry
CHAPTER 7 Submanifold geometry 7.1 Introduction In this chapter, we study the extrinsic geometry of Riemannian manifolds. Historically speaking, the field of Differential Geometry started with the study of curves and surfaces in R3, which originated as a development of the invention of infinitesimal calculus. Later investigations considered arbitrary dimensions and codimensions. Our discussion in this chapter is centered in submanifolds of spaces forms. A most fundamental problem in submanifold geometry is to discover simple (sharp) relationships between intrinsic and extrinsic invariants of a submanifold. We begin this chapter by presenting the standard related results for submanifolds of space forms, with some basic applications. Then we turn to the Morse index theorem for submanifolds. This is a very important theorem that can be used to deduce information about the topology of the submanifold. Finally, we present a brief account of the theory of isoparametric submanifolds of space forms, which in some sense are the submanifolds with the simplest local invariants, and we refer to [BCO16, ch. 4] for a fuller account. 7.2 The fundamental equations of the theory of isometric immersions The first goal is to introduce a number of invariants of the isometric immersion. From the point of view of submanifold geometry, it does not make sense to distinguish between two isometric immersions of M into M that differ by an ambient isometry. We call two isometric immersions f : (M, g) (M, g) and f ′ : (M, g′) (M, g) congruent if there exists an isometry ϕ of M such → → that f ′ = ϕ f. In this case, ◦ g′ = f ′∗g = f ∗ϕ∗g = f ∗g = g.