Sources of Vascular Nitric Oxide and Reactive Oxygen Species and Their Regulation
Total Page:16
File Type:pdf, Size:1020Kb
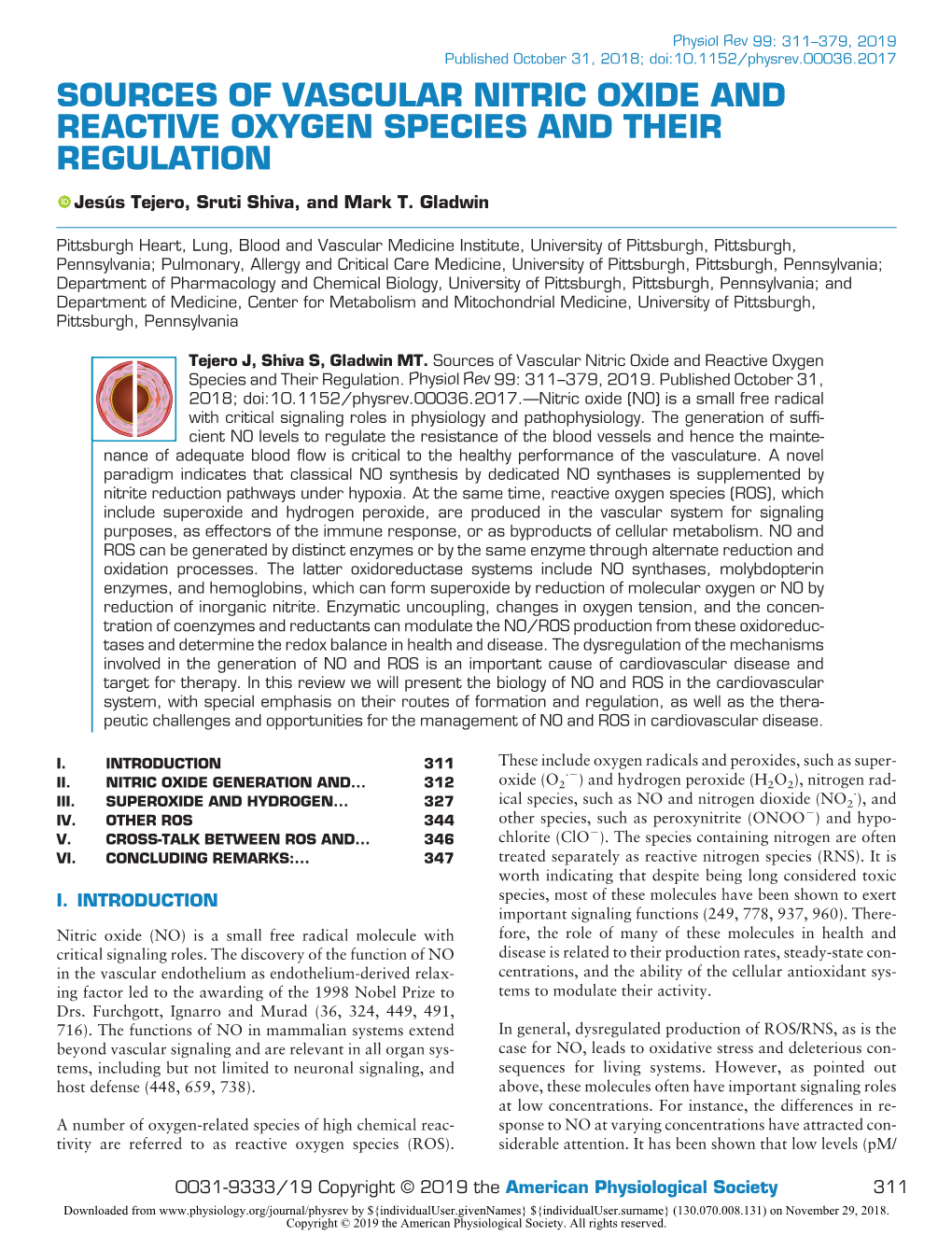
Load more
Recommended publications
-
Greg J Sheehan, FWS Principal Deputy Director
Greg J Sheehan, FWS Principal Deputy Director Tue Jun 6, 2017 2:45pm FWS weekly check in meeting Video call: (b) (5), (b) (6) Where: AS/FWP Conference Room -- 3144 Calendar: Maureen Foster Created by: Tasha Robbins Who: Thomas Irwin, Charisa Morris, Maureen Foster, Richard Goeken, GregSheehan, Wendy Fink, Stephen Guertin, Catherine Gulac, Jason Larrabee,Tasha Robbins, Aurelia Skipwith, Marshall Critchfield, Heather Swift,Jim Kurth, Todd Willens, Roslyn Sellars, Zachariah Gambill, PegRomanik, Barbara Wainman 3pm *FWS weekly check in meeting Video call: (b) (5), (b) (6) Where: AS/FWP Conference Room -- 3144 Calendar: Greg Sheehan Created by: Roslyn Sellars Wed Jun 7, 2017 1pm OIG monthly status update meeting/conference call (Jim, Steve, Kathy Garrity, Keith Toomey, Charisa) Room 3357 Video call: (b) (5), (b) (6) Where: Dial: (b) (5) , Code: (b) (5) Calendar: Jim Kurth Created by: Roslyn Sellars Who: Casey Hammond, Stephen Guertin, Katherine Garrity, Jim Kurth, GregSheehan, Charisa Morris, Keith Toomey 2:30pm [Asst Directors/Regional Directors Only-No Deputies or Actings] - Weekly Directorate VTC: Transition Check-In--Room 3038 Video call: (b) (5), (b) (6) Calendar: Jim Kurth Created by: Thomas Irwin Who: [email protected], Tom Melius, [email protected], WandaCantrell, Edward Grace, [email protected], Henry Schlitzer,Pamela Michalegko, Kenneth Taylor, Benjamin Tuggle, Brian Bloodsworth,Robyn Thorson, Gary Frazer, Michael Gale, Jim Kurth, Charisa Morris,Paul Rauch, Denise Thompson, Seth Mott, Cynthia Martinez,cynthia_dohner@fws -
Obituaries Buffalo News 2010 by Name
Obituaries as found in the Buffalo News: 2010 Date of Place of Date, Page of Last Name/Maiden First Name M.I. Age Death Death/Birth/Residence Date, Page detailed obit Abbarno Vincent "Lolly" A. 9/26/2010 Kenmore, NY 9-30-2010: C4 Abbatte/Saunders Murielle A. 87 1/11/2010 1-13-2010: B4 Abbo Joseph D. 57 5/31/2010 Lewiston, NY 6-3-2010: B4 Brooksville, FL; formerly of Abbott Casimer "Casey" 12/19/22009 Cheektowaga, NY 4-18-2010: C6 Abbott Phillip C. 3/31/2010 4-3-2010: B4 Abbott Stephen E. 7/6/2010 7-8-2010: B4 Abbott/Pfoetsch Barbara J. 4/20/2010 5-2-2010: B4 Abeles Esther 95 1/31/2010 2-4-2010: C4 Abelson Gerald A. 82 2/1/2010 Buffalo, NY 2-3-2010: B4 Abraham Frank J. 94 3/21/2010 3-23-2010: B4 Abrahams/Gichtin Sonia 2/10/2010 died in California 2-14-2010: C4 Abramo Rafeala 93 12/16/2010 12-19-2010: C4 Abrams Charlotte 4/6/2010 4-8-2010: B4 Abrams S. "Michelle" M. 37 5/21/2010 Salamanca, NY 5-23-2010: B4 Abrams Walter I. 5/15/2010 Basom, NY 5-19-2010: B4 Abrosette/Aksterowicz Sister Mary 6/18/2010 6-19-2010: C4 Refer to BEN 2-21-2010: B6/7/8 for more possible Abshagen Charles, Jr. L. 73 2/19/2010 North Tonawanda, NY 2-22-2010: B8 information Acevedo Miguel A. 10/6/2010 Buffalo, NY 10-27-2010: B4 Achkar John E. -
Vol. 83 Wednesday, No. 211 October 31, 2018 Pages 54663–54860
Vol. 83 Wednesday, No. 211 October 31, 2018 Pages 54663–54860 OFFICE OF THE FEDERAL REGISTER VerDate Sep 11 2014 20:07 Oct 30, 2018 Jkt 247001 PO 00000 Frm 00001 Fmt 4710 Sfmt 4710 E:\FR\FM\31OCWS.LOC 31OCWS amozie on DSK3GDR082PROD with FRONT MATTER WS II Federal Register / Vol. 83, No. 211 / Wednesday, October 31, 2018 The FEDERAL REGISTER (ISSN 0097–6326) is published daily, SUBSCRIPTIONS AND COPIES Monday through Friday, except official holidays, by the Office PUBLIC of the Federal Register, National Archives and Records Administration, under the Federal Register Act (44 U.S.C. Ch. 15) Subscriptions: and the regulations of the Administrative Committee of the Federal Paper or fiche 202–512–1800 Register (1 CFR Ch. I). The Superintendent of Documents, U.S. Assistance with public subscriptions 202–512–1806 Government Publishing Office, is the exclusive distributor of the official edition. Periodicals postage is paid at Washington, DC. General online information 202–512–1530; 1–888–293–6498 Single copies/back copies: The FEDERAL REGISTER provides a uniform system for making available to the public regulations and legal notices issued by Paper or fiche 202–512–1800 Federal agencies. These include Presidential proclamations and Assistance with public single copies 1–866–512–1800 Executive Orders, Federal agency documents having general (Toll-Free) applicability and legal effect, documents required to be published FEDERAL AGENCIES by act of Congress, and other Federal agency documents of public Subscriptions: interest. Assistance with Federal agency subscriptions: Documents are on file for public inspection in the Office of the Federal Register the day before they are published, unless the Email [email protected] issuing agency requests earlier filing. -
Elecciones Anuales Grado 2020 − Delegados De Curso Página 1 De 51
Elecciones anuales Grado 2020 − Delegados de curso Fecha de impresión: 27−11−2020 Grado en Ingeniería Eléctrica − Curso: 1 Nº Apellido1 Apellido2 Nombre DNI 1 ARIAS RODRIGUEZ GABRIEL 7***03*3 2 CAMPOS LUCAS ALEJANDRO 7*18***9 3 FERNANDEZ MIERA ADRIAN *2***144 4 GARCÍA GARCÍA IÑAKI 20*0*3** 5 GARCIA HERRANZ JULIO *2*94**8 6 GONZALEZ VENERO HERNAN **08*08* 7 GUERRA SAINZ FRANCISCO RAMON **104**6 8 MADARIAGA GONZALEZ ERNESTO 7*103*** 9 MARCANO CUBILLAS MANUEL 7**2*0*1 10 MAZO LOPEZ PEDRO PABLO DEL **0*26*0 11 MOYA MANTECON LAURA 7*1**15* 12 ORTIZ SAMPERIO VICTOR *21***11 13 PEREDA SOLARES JORGE **1*501* 14 RAMOS MARTINEZ SERGIO 7**8**77 15 RODRIGUEZ EIZAGUIRRE JORGE 7*0**1*8 16 ROJAS LOPEZ FELIPE ***00*03 17 SOLANO RUIZ ALVARO ***0319* 18 XIANG QIXIN ****6291 En cumplimiento de lo dispuesto apartado 1 de la Disposición adicional séptima de la Ley Orgánica 3/2018, de 5 de diciembre, de Protección de Datos Personales y garantía de los derechos digitales, las personas que figuran en este listado aparecen identificadas con cuatro cifras numéricas aleatorias del DNI, NIE, Pasaporte o documento equivalente. En los casos en que el número de estos documentos tenga menos de seis cifras numéricas, se asignará un número aleatorio confeccionado con este fin. Los números aleatorios asignados pueden consultarse en Campus Virtual (Menú general / Datos personales). Página 1 de 51 Elecciones anuales Grado 2020 − Delegados de curso Fecha de impresión: 27−11−2020 Grado en Ingeniería Eléctrica − Curso: 2 Nº Apellido1 Apellido2 Nombre DNI 1 ALONSO MURGA RAUL -
C9orf72 Frontotemporal Dementia and Amyotrophic Lateral Sclerosis: Investigating Repeat Pathology in Cell Culture Models and Human Post-Mortem Brain
C9orf72 frontotemporal dementia and amyotrophic lateral sclerosis: investigating repeat pathology in cell culture models and human post-mortem brain. Charlotte Elizabeth Ridler A thesis submitted in partial fulfilment of the requirements for the degree of Doctor of Philosophy from University College London Department of Neurodegenerative Disease Institute of Neurology University College London 2016 1 Declaration I, Charlotte Ridler, confirm that the work presented in this thesis is my own. Where information has been derived from other sources, I confirm that this has been indicated in the thesis. 2 Acknowledgements My deepest thanks go to everyone who has helped and supported me during this PhD project, without whom it would not have been possible. Thank you firstly to my supervisor Dr. Adrian Isaacs for all of his guidance, encouragement and enthusiastic discussions during this exciting and unpredictable project. Thank you also to Dr. Sarah Mizielinska, who has been an incredible mentor and role-model during this PhD; I have learnt a huge amount from her endless dedication and generosity. I would also like to thank my secondary supervisor Prof. Elizabeth Fisher for all her wise words of advice. Thank you to all members of the Isaacs and Fisher labs past and present. Special thanks to Frances Norona, Dr. Karen Cleverly, Julian Pietrzyk, Dr. Ione Woollacott and Dr. Anny Devoy who were all great help during the cloning phase of this project; to Dr. Rubika Balendra for all her help and discussions in the nucleoli work; and to Dr. Emma Clayton and Dr. Roberto Simone for their constant support. A number of other people have lent me their valuable expertise for this project, for which I am very grateful. -
1¾ « Lõ Ô C:\WORD5\HPLASER
................................................................ INN15 IIIIIIIIIIII NNNN NN NNNN NN 11 555555 II NN NN NN NN NN NN 111 55 II NN NN NN NN NN NN 11 55 II NN NN NN NN NN NN = 11 55555 II NN NN NN NN NN NN = 11 55 II NN NN NN NN NN NN 11 55 55 IIIIIIIIIII NN NNNN NN NNNN 111111 555555 INTERNATIONAL NETWORK NEWSLETTER NUMBER 15 JUNE 1991 ============================================================ ABSTRACT Thanks are expressed to the Gang of Three who so successfully organized the Network's conference in Normal in May....announcement is made of the next Network Graduate Workshop and the next Network International Conference in Milwaukee in 1993 the recipients of the Network's initiative to support graduate student travel are recognized....the procedures for the next Network Dissertation Award are announced....The Committee on the Future of the Network reports on its proposals, along with progress report on the talks on the proposed merger with ISSPR .. a new feature of the Newsletters is announced .... a copy of the program for the Normal conference is included in case those who missed the conference would like to benefit by contacting authors of papers MAJOR ANNOUNCEMENT: IT IS TIME TO RENEW YOUR MEMBERSHIP. NOTE THAT YOU CAN GET A DISCOUNT BY RENEWING BEFORE SEPTEMBER 30TH THE "NORMAL" CONFERENCE The Normal conference was, from start to finish, a thoroughly well-organized, thoroughly stimulating and thoroughly enjoyable affair with a wonderful atmosphere free from all rancor and strife! Sound too good to be true? Well, if you were not there then ask someone who was and if you were, you probably have already told others. -
University Staff University Staff
www.ualberta.ca UNIVERSITY OF ALBERTA University Staff University University Staff In Memoriam: Agrawal, Babita, BSc MSc (Allahabad), PhD Anderson, Colin C, BSc (Honours) PhD (Western Al-Hussaini, Ata N, BSc (Iraq), MSc (Purdue), PhD (Illinois), Professor Emeritus of Mathematical Sciences (Alberta), Assistant Professor of Surgery (2001, Ontario), Assistant Professor of Surgery (2002, (1968, 1999). 2001). 2002). Bennett, Bonnie L, Manager, Admin Services and Information Systems, Human Resources (1998, 1998). Aguerrevere, Felipe L, BS (Simon Bolivar), Anderson, Todd W, Director of the Bookstore Blackley, Frank D, MA PhD (Toronto), Professor Emeritus of History (1950, 1983). MAdmin (IESA), PhD (Calif-LA), Assistant (1999, 2001). Chamberlin, Charles R, BA (Iowa), MA (Minnesota), PhD (Minnesota), Professor Emeritus of Elementary Professor of Finance & Management Science Anderson, Wayne, Administrative Professional Education (1969, 1995). (2000, 2000). Officer in the Bookstore (1999, 1999). Faulds, Alexander G, MCSP DipTP (London), Professor Emeritus of Physical Therapy (1958, 1977). Ahmed, Syed N, MBBS (Dow Medical), Assistant Andrew, Susan E, BSc (Toronto), MSc (Simon Ferrate, Juan, Lic (Barcelona), Professor Emeritus of Comparative Literature (1962, 1985). Professor of Medicine (2002, 2002). Fraser), PhD (British Columbia), Assistant Gifford, David J, BSc PhD (Alberta), Professor of Biological Sciences (1986, 1998). Albrecht, G Diane, BComm (Alberta), Director of Professor of Medical Genetics (1998, 1998). Gilbert, James AL, MB ChB MD FRCP (Edinburgh), FRCP(C), FRCP (London), FACP, Professor Emeritus of Staff Programs in Human Resources (2000, Andrews, Debra, BSC (Wilkes), MD (Harvard), Medicine (1950, 1983). 2001). FRCP, Associate Professor of Pediatrics (1987, Gunning, Harry E, BA MA PhD (Toronto), DSc (Guelph), DSc (Queen’s), FRSC, Professor Emeritus of Chemistry Alexander, Scott DB, BSc MSc PhD (Alberta), 2003). -
Surname Given Age Date Page Maiden Note Aageberg Alice F. 101 11-Feb D-1 See Also Article Feb. 12, P. C-5 Abegg Frances J. 64 9
Surname Given Age Date Page Maiden Note Aageberg Alice F. 101 11-Feb D-1 See also article Feb. 12, p. C-5 Abegg Frances J. 64 9-Jun D-1 Abel Delores Ruth 57 9-Sep B-6 Abel Ralph 31-Dec D-5 Abell Thelma 80 18-Dec A-11 See article, p. A-11 Abney Raymond 83 15-Dec D-1 See article, p. D-1 Acheson Helen G. 61 26-Apr E-2 Adamczyk Richard J. 48 5-Nov C-10 Adamovich Michael 77 10-Feb D-1 Adams Altha B. 91 12-Jan C-2 Adams Corinne, Deputy 79 12-Mar C-3 See also article March 13, p. C-1 Adams Frances D. 53 10-Nov D-5 Malloy Adams Leonard Eugene 58 2-Jun D-3 (Preacher) Adams Marie Mae 75 10-Mar C-1 Adams Robert F. 72 11-Jan D-1 Adams Susan L. 35 3-Mar C-6 Adams Thomas A. 12 Februray 2 A-6 Adams Thomas P. 38 19-Jan A-8 Addison James Grover "J. G." 69 3-Apr C-1 Adelsperger Edward H. 69 25-Mar D-1 Adelsperger Helen 71 24-Nov B-5 Adkins Kathleen 70 3-Feb B-7 Adler Sophie 74 4-Feb D-1 Adolph Bernard 60 2-Oct C-2 Adzima Rose 26-May D-5 Kubeck Agee Hardy 66 13-Jan A-1 See article, p. A-1 Agerter Tim D. 22 19-May D-1 See also article, p. A-2 Ahearn Mary 82 22-Apr C-3 Doolin Akhtar Julie Wayman 26 15-Apr C-5 Alaimo Bartole (Bart) 66 24-Oct B-2 Alaimo Damiana 86 20-Jan C-5 Alarcon Damiana 89 26-Jun C-1 Albert Florence E. -
Climate Change Effects on North American Inland Fish Populations and Assemblages
Fisheries ISSN: 0363-2415 (Print) 1548-8446 (Online) Journal homepage: http://www.tandfonline.com/loi/ufsh20 Climate Change Effects on North American Inland Fish Populations and Assemblages Abigail J. Lynch, Bonnie J. E. Myers, Cindy Chu, Lisa A. Eby, Jeffrey A. Falke, Ryan P. Kovach, Trevor J. Krabbenhoft, Thomas J. Kwak, John Lyons, Craig P. Paukert & James E. Whitney To cite this article: Abigail J. Lynch, Bonnie J. E. Myers, Cindy Chu, Lisa A. Eby, Jeffrey A. Falke, Ryan P. Kovach, Trevor J. Krabbenhoft, Thomas J. Kwak, John Lyons, Craig P. Paukert & James E. Whitney (2016) Climate Change Effects on North American Inland Fish Populations and Assemblages, Fisheries, 41:7, 346-361, DOI: 10.1080/03632415.2016.1186016 To link to this article: http://dx.doi.org/10.1080/03632415.2016.1186016 Published online: 29 Jun 2016. Submit your article to this journal Article views: 87 View related articles View Crossmark data Full Terms & Conditions of access and use can be found at http://www.tandfonline.com/action/journalInformation?journalCode=ufsh20 Download by: [University of Missouri-Columbia] Date: 02 July 2016, At: 10:48 FEATURE Climate Change Effects on North American Inland Fish Populations and Assemblages Abigail J. Lynch U. S. Geological Survey (USGS), National Climate Change and Wildlife Science Center, 12201 Sunrise Valley Drive, MS-516, Reston, VA 20192. E-mail: [email protected] Bonnie J. E. Myers USGS, National Climate Change and Wildlife Science Center, Reston, VA Cindy Chu Ontario Ministry of Natural Resources and Forestry, Aquatic Research and Monitoring Section, Peterborough, ON, Canada Lisa A. Eby Wildlife Biology Program, College of Forestry and Conservation, University of Montana, Missoula, MT Jeffrey A. -
Surname Given Maiden Name Date Page Adams Floyd, O. 20-Feb-48 43 Adams John, Myron 30-May-48 46 Adley Joseph, A
Surname Given Maiden Name Date Page Adams Floyd, O. 20-Feb-48 43 Adams John, Myron 30-May-48 46 Adley Joseph, A. 26-May-48 64 Agles Carolyn Foust 23-Dec-48 50 Alcantar Nellie 19-Nov-48 28 Alger William Henry 23-Feb-48 87 Allen Francis 8-Apr-48 72 Allen Louise 2-Feb-48 76 Almason Catherine May l8, 1948 69 Almay Edward 20-Dec-48 54 Alsman Marion, E. 24-Oct-48 44 Alt Henry, C. April ll, 1948 66 Alvarez Ferdinand, J. (S/Sgt.) December 2l, 1948 25 Alyea William November l5, 1948 77 Ambrus John D. (Pfc.) August l7, 1948 26 Amrai Katherine November l0, 1948 78 Ancich John 27-May-48 65 Anderson Arvid Carl III 23-Aug-48 6 weeks Anderson Bertie Marie 5-Sep-48 2 months Anderson George (Tech/Sgt.) December l6, 1948 29 Anderson John March 2l, 1948 66 Andreotti Peter (2nd Lt.) 28-Jun-48 24 Androff Thomas Carl 24-Oct-48 3 Anest Harry 29-Aug-48 67 Angelcoff George March l5, 1948 46 Angelich Emil February l6, 1948 58 Antonowicz Joseph, J. 9-May-48 70 Aponiak Alex 6-Jan-48 68 Armstrong Myrtle 22-Mar-48 66 Armstrong Walter, S. March l0, 1948 51 Arsulich Thomas 23-Nov-48 77 Augustyn Louis Septermber l9, 1948 57 Ault Lulu 30-Nov-48 75 Austgen Lillian 2-May-48 68 Austgen Mary 22-Jun-48 66 Baars William 6-Jan-48 83 Babbitt Nellie 4-May-48 76 Babe Henry, O. 23-Feb-48 58 Babic John 26-Jan-48 58 Babicz Louis 29-Jul-48 60 Babincsak Elizabeth 29-Aug-48 81 Babinscak Michael 7-Jun-48 64 Babyak Michael 27-Feb-48 58 Bacan Nick August l6, 1948 53 Baert Jennie 30-Aug-48 74 Bagamery James 8-Dec-48 69 Bailey Maude November l, 1948 66 Baker Gertrude Ausugst 25, 1948 86 Baker Ima December l5, 1948 46 Baker Lloyd Ira 7-Dec-48 63 Balczo James 23-Nov-48 5 months Baliga Mathew July l9, 1948 53 Ballon Albert, J. -
Active Attorneys
Current as of: 9/28/2021 5:46:00 AM Active Attorneys Attorney Name Bar Admission Date ECF Filing Status Kaakarli, Hasan 2/3/2017 Has an ECF Account Kaake, Andrew, R. 2/17/2004 Has an ECF Account Kaanta, Leonard, A. 2/4/2003 Needs an ECF Account Kaare, Linda, Sue 2/21/1991 Needs an ECF Account Kaas, Sarah, F. 3/14/2003 Needs an ECF Account Kaatz, Robert, A. 4/14/2004 Needs an ECF Account Kab, Marina 7/24/2007 Has an ECF Account Kabalka, Stephen, G. 11/18/1998 Needs an ECF Account Kabat, Alan, R. 7/9/2001 Has an ECF Account Kabat, Andrew, A. 12/14/1994 Has an ECF Account Kabat, Michael, D. 11/26/2012 Has an ECF Account Kabat, Patrick, Stephen 5/21/2018 Has an ECF Account Kabb, Kenneth, S. 8/1/1983 Needs an ECF Account Kable, Kenneth, W. 3/20/1995 Needs an ECF Account Kacena, James, L. 2/7/1983 Needs an ECF Account Kacenjar, Allen, A. 4/13/2007 Has an ECF Account Kachoyeanos, Janet, A. 1/21/2003 Needs an ECF Account Kachur, Courtney, A. 9/23/2005 Needs an ECF Account Kackley, Debra, L. 11/6/1992 Needs an ECF Account Kacprowski, Nickolas, A. 11/27/2007 Needs an ECF Account Kaczala, Larry, A. 9/10/1987 Needs an ECF Account Kaczka, Michael, J. 7/19/2004 Has an ECF Account Kaczmarczyk, Sandra 8/31/2007 Needs an ECF Account Kaczmarek, William, F. 4/17/1990 Needs an ECF Account Kaczor, David, L. -
List of Participants for the 19 OSCE Ministerial Council Meeting 6 – 7
MC.INF/15/12/Rev.5 6 December 2012 ENGLISH only List of Participants for the 19th OSCE Ministerial Council Meeting 6 – 7 December 2012 DUBLIN 19th MEETING OF THE MINISTERIAL COUNCIL 6 - 7 December 2012 DUBLIN DELEGATION SURNAME FIRST NAME FUNCTION ALBANIA PANARITI Edmond Minister of Foreign Affairs of Albania ALBANIA MELO Ilir Director General Global Issues and International Organizations ALBANIA SKAPI Armand Chief of Staff of the MFA of Albania ALBANIA KOCI Spiro Ambassador, Permanent Representative ALBANIA SAKIQI Xhodi Counsellor, Permanent Mission of Albania GERMANY LINK Mic hael Georg Minister of State, Federal Foreign Office GERMANY LÜDEKING Rüdiger Ambassador, Head of the Permanent Mission to the OSCE GERMANY SALBER Herbert Deputy Political Director, Federal Foreign Office GERMANY SCHMUNK Michael Deputy Head of Permanent Mission to the OSCE GERMANY SCHULTZE Eberhard Head of OSCE Division, Federal Foreign Office GERMANY WOLTER Detlef Head of Division, Conventional Arms Control and CSBMs, Federal Foreign Office GERMANY PRADEL Hans-Henning Senior Military Adviser, Permanent Mission to the OSCE GERMANY PETRY Uwe Counsellor, Permanent Mission to the OSCE GERMANY HOVORKA Uwe Military Adviser, Permanent Mission to the OSCE GERMANY HOFMEISTER Manfred Military Adviser, Permanent Mission to the OSCE GERMANY PRESCHER Jörg Military Adviser, Permanent Mission to the OSCE GERMANY JANTSCH Heike Counsellor, Permanent Mission to the OSCE GERMANY WAGNER-MITCHELL Anne Counsellor, Permanent Mission to the OSCE GERMANY KAHRL Julia Assistant to Minister