Surface Enhanced Raman Scattering Enhancement Factors: a Comprehensive Study
Total Page:16
File Type:pdf, Size:1020Kb
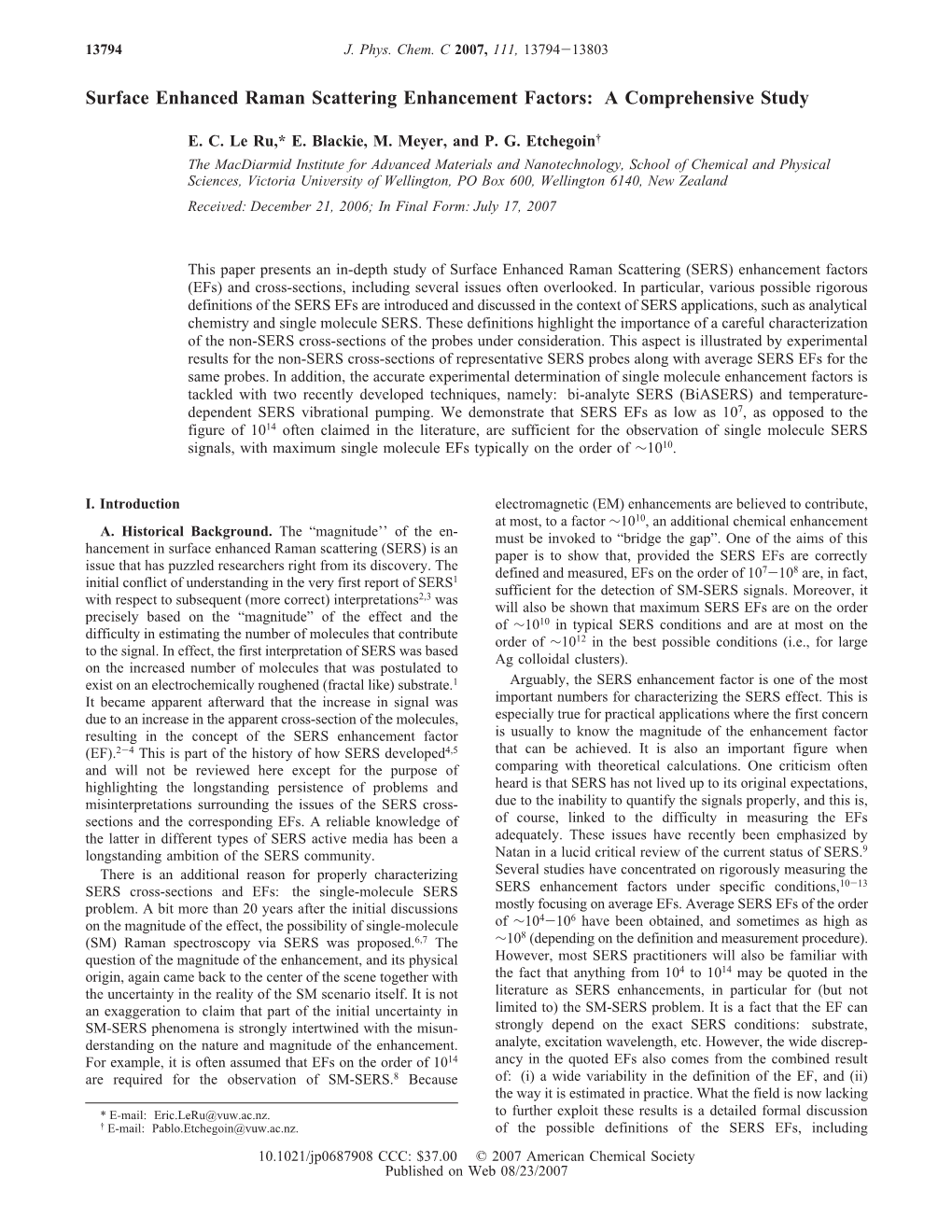
Load more
Recommended publications
-
Polarization- and Angular-Resolved Optical Response of Molecules on Anisotropic Plasmonic Nanostructures
nanomaterials Review Polarization- and Angular-Resolved Optical Response of Molecules on Anisotropic Plasmonic Nanostructures Martin Šubr * and Marek Procházka * ID Faculty of Mathematics and Physics, Institute of Physics, Charles University, 121 16 Prague 2, Czech Republic * Correspondence: [email protected] (M.S.); [email protected] (M.P.); Tel.: +420-221-911-463 (M.S.); +420-221-911-474 (M.P.) Received: 10 May 2018; Accepted: 7 June 2018; Published: 9 June 2018 Abstract: A sometimes overlooked degree of freedom in the design of many spectroscopic (mainly Raman) experiments involve the choice of experimental geometry and polarization arrangement used. Although these aspects usually play a rather minor role, their neglect may result in a misinterpretation of the experimental results. It is well known that polarization- and/or angular- resolved spectroscopic experiments allow one to classify the symmetry of the vibrations involved or the molecular orientation with respect to a smooth surface. However, very low detection limits in surface-enhancing spectroscopic techniques are often accompanied by a complete or partial loss of this detailed information. In this review, we will try to elucidate the extent to which this approach can be generalized for molecules adsorbed on plasmonic nanostructures. We will provide a detailed summary of the state-of-the-art experimental findings for a range of plasmonic platforms used in the last ~15 years. Possible implications on the design of plasmon-based molecular sensors for maximum signal enhancement will also be discussed. Keywords: SERS; Raman; plasmonics; metallic nanostructures; polarization and angular dependences; molecular orientation; ellipsometry 1. Introduction Methods of optical spectroscopy, such as absorption spectroscopy, Raman scattering spectroscopy or fluorescence spectroscopy, can be performed in a wide range of experimental setups relying on a proper choice of instrumentation, light sources, waveguides, monochromators, detectors etc. -
Raman Spectra of Methane, Ethylene, Ethane, Dimethyl Ether, Formaldehyde and Propane for Combustion Applications
Raman Spectra of Methane, Ethylene, Ethane, Dimethyl ether, Formaldehyde and Propane for Combustion Applications Item Type Article Authors Magnotti, G.; KC, Utsav; Varghese, P.L.; Barlow, R.S. Citation Raman Spectra of Methane, Ethylene, Ethane, Dimethyl ether, Formaldehyde and Propane for Combustion Applications 2015 Journal of Quantitative Spectroscopy and Radiative Transfer Eprint version Post-print DOI 10.1016/j.jqsrt.2015.04.018 Publisher Elsevier BV Journal Journal of Quantitative Spectroscopy and Radiative Transfer Rights NOTICE: this is the author’s version of a work that was accepted for publication in Journal of Quantitative Spectroscopy and Radiative Transfer. Changes resulting from the publishing process, such as peer review, editing, corrections, structural formatting, and other quality control mechanisms may not be reflected in this document. Changes may have been made to this work since it was submitted for publication. A definitive version was subsequently published in Journal of Quantitative Spectroscopy and Radiative Transfer, 8 May 2015. DOI: 10.1016/ j.jqsrt.2015.04.018 Download date 04/10/2021 05:45:56 Link to Item http://hdl.handle.net/10754/552799 Author's Accepted Manuscript Raman Spectra of Methane, Ethylene, Ethane, Dimethyl ether, Formaldehyde and Propane for Combustion Applications G. Magnotti, U. KC, P.L. Varghese, R.S. Barlow www.elsevier.com/locate/jqsrt PII: S0022-4073(15)00169-7 DOI: http://dx.doi.org/10.1016/j.jqsrt.2015.04.018 Reference: JQSRT4982 To appear in: Journal of Quantitative Spectroscopy & Radiative Transfer Received date: 21 January 2015 Revised date: 27 April 2015 Accepted date: 28 April 2015 Cite this article as: G. -
Quantitative Analysis of Light Scattering in Polarization-Resolved Nonlinear Microscopy Hilton B
Quantitative analysis of light scattering in polarization-resolved nonlinear microscopy Hilton B. de Aguiar, Paulina Gasecka, Sophie Brasselet To cite this version: Hilton B. de Aguiar, Paulina Gasecka, Sophie Brasselet. Quantitative analysis of light scattering in polarization-resolved nonlinear microscopy. Optics Express, Optical Society of America - OSA Publishing, 2015, 23, pp.8960-8973. 10.1364/OE.23.008960. hal-01216129 HAL Id: hal-01216129 https://hal-amu.archives-ouvertes.fr/hal-01216129 Submitted on 15 Oct 2015 HAL is a multi-disciplinary open access L’archive ouverte pluridisciplinaire HAL, est archive for the deposit and dissemination of sci- destinée au dépôt et à la diffusion de documents entific research documents, whether they are pub- scientifiques de niveau recherche, publiés ou non, lished or not. The documents may come from émanant des établissements d’enseignement et de teaching and research institutions in France or recherche français ou étrangers, des laboratoires abroad, or from public or private research centers. publics ou privés. Quantitative analysis of light scattering in polarization-resolved nonlinear microscopy Hilton B. de Aguiar,1,∗ Paulina Gasecka1 and Sophie Brasselet1,2 1Aix-Marseille Universite,´ CNRS, Centrale Marseille, Institut Fresnel UMR 7249, 13013 Marseille, France [email protected] ∗[email protected] Abstract: Polarization resolved nonlinear microscopy (PRNM) is a powerful technique to gain microscopic structural information in biological media. However, deep imaging in a variety of biological specimens is hindered by light scattering phenomena, which not only degrades the image quality but also affects the polarization state purity. In order to quantify this phenomenon and give a framework for polarization resolved microscopy in thick scattering tissues, we develop a characterization methodology based on four wave mixing (FWM) process. -
An Empirical Approach to the Bond Additivity Model in Quantitative
An Empirical Approach to the Bond Additivity Model in Quantitative Interpretation of Sum Frequency Generation Vibrational Spectra Hui Wua†, Wen-kai Zhangb†, Wei Ganb, Zhi-feng Cuia, and Hong-fei Wanga,b∗ a. Department of Physics, Anhui Normal University, Wuhu, Anhui Province, China 241000; b. State Key Laboratory of Molecular Reaction Dynamics, Institute of Chemistry, the Chinese Academy of Sciences, Beijing, China, 100080 (Dated: October 29, 2018) Knowledge of the ratios between different polarizability βi′j′k′ tensor elements of a chemical group in a molecule is crucial for quantitative interpretation and polarization analysis of its SFG- VS spectrum at interfaces. The bond additivity model or the hyperpolarizability derivative model along with experimentally obtained Raman depolarization ratios has been widely used to obtain such tensor ratios for the CH3, CH2, and CH groups. Successfully, such treatment can quantitatively reproduce the intensity polarization dependence in SFG-VS spectra for the symmetric (ss) and asymmetric (as) stretching modes of CH3 and CH2 groups, respectively. However, the relative intensity between the ss and as modes usually does not agree with each other within this model even for some of the simplest molecular systems, such as the air/methanol interface. This fact certainly has cast uncertainties on the effectiveness and conclusions based on the bond additivity model. One of such examples is that the as mode of CH3 group has never been observed in SFG- VS spectra from the air/methanol interface, while this as mode is usually very strong for SFG-VS spectra from the air/ethanol, other short chain alcohol, as well as long chain surfactants, interfaces. -
Article Depolar- Back-Trajectory Analysis Was Performed for the Air Mass Over Ization Ratio at 532 Nm for This Session
Atmos. Meas. Tech., 12, 119–128, 2019 https://doi.org/10.5194/amt-12-119-2019 © Author(s) 2019. This work is distributed under the Creative Commons Attribution 4.0 License. Profiling of CH4 background mixing ratio in the lower troposphere with Raman lidar: a feasibility experiment Igor Veselovskii1, Philippe Goloub2, Qiaoyun Hu2, Thierry Podvin2, David N. Whiteman3, Mikhael Korenskiy1, and Eduardo Landulfo4 1Physics Instrumentation Center of General Physics Institute, Troitsk, Moscow, Russia 2Laboratoire d’Optique Atmosphérie, Université de Lille-CNRS, Villeneuve-d’Ascq, France 3NASA Goddard Space Flight Center, Greenbelt, USA 4Instituto de Pesquisas Energeticas e Nucleares, São Paulo, Brazil Correspondence: Igor Veselovskii ([email protected]) Received: 3 September 2018 – Discussion started: 20 September 2018 Revised: 7 December 2018 – Accepted: 12 December 2018 – Published: 7 January 2019 Abstract. We present the results of methane profiling in 1 Introduction the lower troposphere using LILAS Raman lidar from the Lille University observatory platform (France). The lidar is Raman spectroscopy is a powerful technique for identifica- based on a frequency-tripled Nd:YAG laser, and nighttime tion of different gases in the atmosphere and for the estima- profiling up to 4000 with 100 m height resolution is possi- tion of their concentration (Weber, 1979), which can be used ble for methane. Agreement between the measured photon- in conjunction with lidar technology (Inaba and Kobayasi, counting rate in the CH4 Raman channel in the free tropo- 1972). An example of such synergy is the Raman lidar for sphere and numerical simulations for a typical CH4 back- water vapor monitoring (Whiteman et al., 1992). -
Raman Optical Activity: a Novel Version of Chiroptical Spectroscopy
CORE Metadata, citation and similar papers at core.ac.uk Provided by IACS Institutional Repository Indian J. Phys. 82(8) 987-1001(2008) Iw Raman optical activity: a novel version of chiroptical spectroscopy Moitrayee Mukherjee, Anamika Mukhopadhyay and Tapas Chakraborty* Department of Physical Chemistry, Indian Association for the Cultivation of Science, Jadavpur, Kolkata-700 032, India E-mail : [email protected] Abstract : We present here an introductory review of Raman optical activity (ROA), a newly developed version of Raman spectroscopy suitable for predicting the absolute configuration of chiral molecules. The phenomenon involves minute differential scattering of the left and right circularly polarized lights from the chiral molecules, compared to the parent Raman scattering the ROA process is weaker by nearly three to five orders of magnitude. An operational theory of the phenomenon is presented with working equations. The connection between the experimentally observed quantities and the molecular parameters of interests is established. A brief description of the layout of the apparatus currently used for ROA measurements is presented along with a concise review of selected studies highlighting the possibilities of potential applications of this new version of chiroptical spectroscopy in the analysis of structural features of chiral molecules. Keywords : Raman scattering, molecular vibrations, optical activity, circular dichroism, chiral molecules, absolute configurations PACSNo. : 33.20.Fb 1. Introduction Polarization is an intrinsic attribute of Raman scattered light {1]. In early days of the discovery, the so called inelastically scattered Stokes shifted 'feeble fluorescence' noticed by Sir C V Raman and co-workers when dry and pur* glycerine was irradiated by ultraviolet light appeared to be strongly polarized [2]. -
Raman Depolarization Ratio of Liquids Probed by Linear Polarization Coherent Anti-Stokes Raman Spectroscopy F
Research Article Received: 10 October 2008 Accepted: 9 February 2009 Published online in Wiley Interscience: (www.interscience.wiley.com) DOI 10.1002/jrs.2289 Raman depolarization ratio of liquids probed by linear polarization coherent anti-Stokes Raman spectroscopy F. Munhoz, S. Brustlein, D. Gachet†, F. Billard‡, S. Brasselet and H. Rigneault∗ We present a simple analytical model to describe the linear polarization coherent anti-Stokes Raman scattering (CARS) spectroscopy. In this scheme, the pump and Stokes beams can have arbitrary linear polarization states and the CARS emitted signal is analyzed along two perpendicular directions. We concentrate on isotropic media without electronic resonances and show that only two polarization CARS measurements performed at the peak and the dip of a CARS spectrum are sufficient to deduce the Raman depolarization ratio, the resonant versus nonresonant contribution, the Raman resonance frequency and the linewidth. We demonstrate the applicability of our scheme to toluene and cyclohexane solutions. Copyright c 2009 John Wiley & Sons, Ltd. Keywords: CARS; polarization; spectroscopy; microscopy Introduction Although we are interested in CARS micro-spectroscopy, we present here a simple approach where we neglect the spatial Among nonlinear optical processes, the specificity of the coherent confinement of the pump and Stokes focused beams. This crude anti-Stokes Raman scattering (CARS) is its coherent and resonant approximation leads to a complete analytic description of the [1] nature. The interaction of the incident fields with the sample spectral polarized CARS signal, including the ratio between the induces a third-order nonlinear polarization, whose properties resonant and nonresonant contributions. Careful inspection of depend on the vector nature and frequencies of the incident the outcomes shows that performing only two measurements electromagnetic fields, as well as the structure of the medium. -
Managing Light Polarization Via Plasmon–Molecule Interactions Within an Asymmetric Metal Nanoparticle Trimer
Managing light polarization via plasmon–molecule interactions within an asymmetric metal nanoparticle trimer Timur Shegai*†, Zhipeng Li†‡, Tali Dadosh§, Zhenyu Zhang¶ʈ, Hongxing Xu‡**††, and Gilad Haran*†† *Department of Chemical Physics, Weizmann Institute of Science, Rehovot 76100, Israel; ‡Beijing National Laboratory for Condensed Matter Physics, Institute of Physics, Chinese Academy of Sciences, Beijing 100080, China; §Department of Condensed Matter Physics, Weizmann Institute of Science, Rehovot 76100, Israel; ¶Materials Science and Technology Division, Oak Ridge National Laboratory, Oak Ridge, TN 37831; ʈDepartment of Physics and Astronomy, University of Tennessee, Knoxville, TN 37996-1200; and **Division of Solid State Physics, Lund University, Box 118, S-22100 Lund, Sweden Communicated by E. Ward Plummer, The University of Tennessee, Knoxville, TN, August 26, 2008 (received for review May 10, 2008) The interaction of light with metal nanoparticles leads to novel a scanning tip was used to flip the fluorescence polarization of phenomena mediated by surface plasmon excitations. In this article single molecules between two directions, in-plane or out-of- we use single molecules to characterize the interaction of surface plane. plasmons with light, and show that such interaction can strongly Here, we employ Raman-scattered light from individual mol- modulate the polarization of the emitted light. The simplest ecules to probe the response of asymmetric nanoparticle aggre- nanostructures that enable such polarization modulation are asym- gates over a range of wavelengths, and to show that these metric silver nanocrystal trimers, where individual Raman scatter- aggregates can dramatically modulate the polarization of the ing molecules are located in the gap between two of the nano- emitted light. -
On the SERS Depolarization Ratio
Nanospectroscopy 2015; 1: 26–32 Research Article Open Access Antonino Foti, Cristiano D’Andrea, Elena Messina, Alessia Irrera, Onofrio M. Maragò, Barbara Fazio, Pietro G. Gucciardi* On the SERS depolarization ratio Abstract: The Raman depolarization ratio is a quantity According to the E4 model,[18-24] in SERS the that can be easily measured experimentally and offers nanoantenna plays a twofold role: firstly it amplifies the unique information on the Raman polarizability tensor local field (excitation-field enhancement) confining it to of molecular vibrations. In Surface Enhanced Raman nanoscale regions (hot spots), and secondly it magnifies Scattering (SERS), molecules are near-field coupled with the Raman scattering (re-radiation enhancement). optical nanoantennas and their scattering properties Molecules lying in the hot spots (located at the edges of are strongly affected by the radiation patterns of the individual nanoantennas or in the nanocavities between nanoantenna. The polarization of the SERS photons is near-field coupled NPs[15,25-29]) experience an amplified consequently modified, affecting, in a non trivial way, the local field and an enhanced re-radiation whenever both measured value of the SERS depolarization ratio. In this the wavelengths of the laser pump (λL) and of the induced article we elaborate a model that describes how the SERS Raman dipole (λR) are close to the LSPR wavelength (λLSPR) depolarization ratio is influenced by the nanoantenna [24]. It is well known that the enhanced local field is re-radiation properties, suggesting how to retrieve polarization sensitive. The only component of the incident information on the Raman polarizability from SERS field yielding the local field amplification is the one experiments. -
Surface Enhanced Raman Scattering Polarization and Angular
www.nature.com/scientificreports OPEN Anisotropic Optical Response of Silver Nanorod Arrays: Surface Enhanced Raman Scattering Received: 28 December 2016 Accepted: 17 May 2017 Polarization and Angular Published: xx xx xxxx Dependences Confronted with Ellipsometric Parameters Martin Šubr1, Martin Petr2, Ondřej Kylián2, Josef Štěpánek1, Martin Veis1 & Marek Procházka1 Silver nanorod arrays prepared by oblique angle deposition (AgOADs) represent versatile, simple and inexpensive substrates for high sensitivity surface enhanced Raman scattering (SERS) applications. Their anisotropic nature suggests that their optical responses such as the SERS signal, the depolarization ratio, reflectivity and ellipsometric parameters critically depend on the states of polarization, nanorod angular arrangement and specific illumination-observation geometry. SERS polarization and angular dependences of AgOADs were measured using methylene blue (MB) molecule. Our study constitutes, to our knowledge, the most detailed investigation of such characteristics of plasmonic nanostructures to date. This is due to the 90°-scattering geometry used in which two out of three Euler angles determining the nanorod spatial orientation and four polarization combinations can be varied simultaneously. We attributed the anisotropic optical response to anisotropic (pseudo) refractive index caused by different periodicity of our structures in different directions since the plasmonic properties were found rather isotropic. For the first time we demonstrate very good correspondence -
Arxiv:1611.06252V2 [Physics.Chem-Ph] 2 Mar 2017 [5]
Compact and versatile laser system for polarization-sensitive stimulated Raman spectroscopy Hugo Kerdoncuff,1, ∗ Mark R. Pollard,1 Philip G. Westergaard,1, 2 Jan C. Petersen,1 and Mikael Lassen1 1Danish Fundamental Metrology, Matematiktorvet 307, DK-2800 Kgs. Lyngby, Denmark 2Current address: OFS Fitel Denmark ApS, Priorparken 680, DK-2605 Brøndby, Denmark We demonstrate a compact and versatile laser system for stimulated Raman spectroscopy (SRS). The system is based on a tunable continuous wave (CW) probe laser combined with a home-built semi-monolithic nanosecond pulsed pump Nd:YVO4 laser at 1064 nm. The CW operation of the probe laser offers narrow linewidth, low noise and the advantage that temporal synchronization with the pump is not required. The laser system enables polarization-sensitive stimulated Raman spectroscopy (PS-SRS) with fast high resolution measurement of the depolarization ratio by simul- taneous detection of Raman scattered light in orthogonal polarizations, thus providing information about the symmetry of the Raman-active vibrational modes. Measurements of the depolarization ratios of the carbon-hydrogen (CH) stretching modes in two different polymer samples in the spec- tral range of 2825{3025 cm−1 were performed. Raman spectra are obtained at a sweep rate of 20 nm/s (84 cm−1/s) with a resolution of 0.65 cm−1. A normalization method is introduced for the direct comparison of the simultaneously acquired orthogonal polarized Raman spectra. I. INTRODUCTION nant background arising from nonresonant contributions to the nonlinear optical response, which alters the Ra- There is a growing demand for novel compact laser sys- man spectra. This background is absent from SRS sig- tems for vibrational spectroscopy and microscopy tools, nals, thus SRS provides an advantage over CARS. -
Download Author Version (PDF)
Analyst Accepted Manuscript This is an Accepted Manuscript, which has been through the Royal Society of Chemistry peer review process and has been accepted for publication. Accepted Manuscripts are published online shortly after acceptance, before technical editing, formatting and proof reading. Using this free service, authors can make their results available to the community, in citable form, before we publish the edited article. We will replace this Accepted Manuscript with the edited and formatted Advance Article as soon as it is available. You can find more information about Accepted Manuscripts in the Information for Authors. Please note that technical editing may introduce minor changes to the text and/or graphics, which may alter content. The journal’s standard Terms & Conditions and the Ethical guidelines still apply. In no event shall the Royal Society of Chemistry be held responsible for any errors or omissions in this Accepted Manuscript or any consequences arising from the use of any information it contains. www.rsc.org/analyst Page 1 of 7 Please do notAnalyst adjust margins 1 2 3 4 Analyst 5 6 7 8 COMMUNICATION 9 10 11 12 Quantitative enantioselective Raman spectroscopy 13 14 J. Kiefera,b,c 15 Received 00th January 20xx, 16 Accepted 00th January 20xx 17 DOI: 10.1039/x0xx00000x 18 19 www.rsc.org/ 20 21 Analytical methods for quantitative enantioselective measurements are identical physicochemical properties. Methods capable of 22 highly desirable in the life sciences. Existing technologies have differentiating enantiomers include NMR spectroscopy,4 23 disadvantages such as limited temporal resolution, the need for molecular microwave5 and fluorescence6 spectroscopy, vibrational circular 24 labeling, or high experimental complexity.