Formation of Dicentric and Acentric Chromosomes, by a Template Switch Mechanism, in Budding Yeast
Total Page:16
File Type:pdf, Size:1020Kb
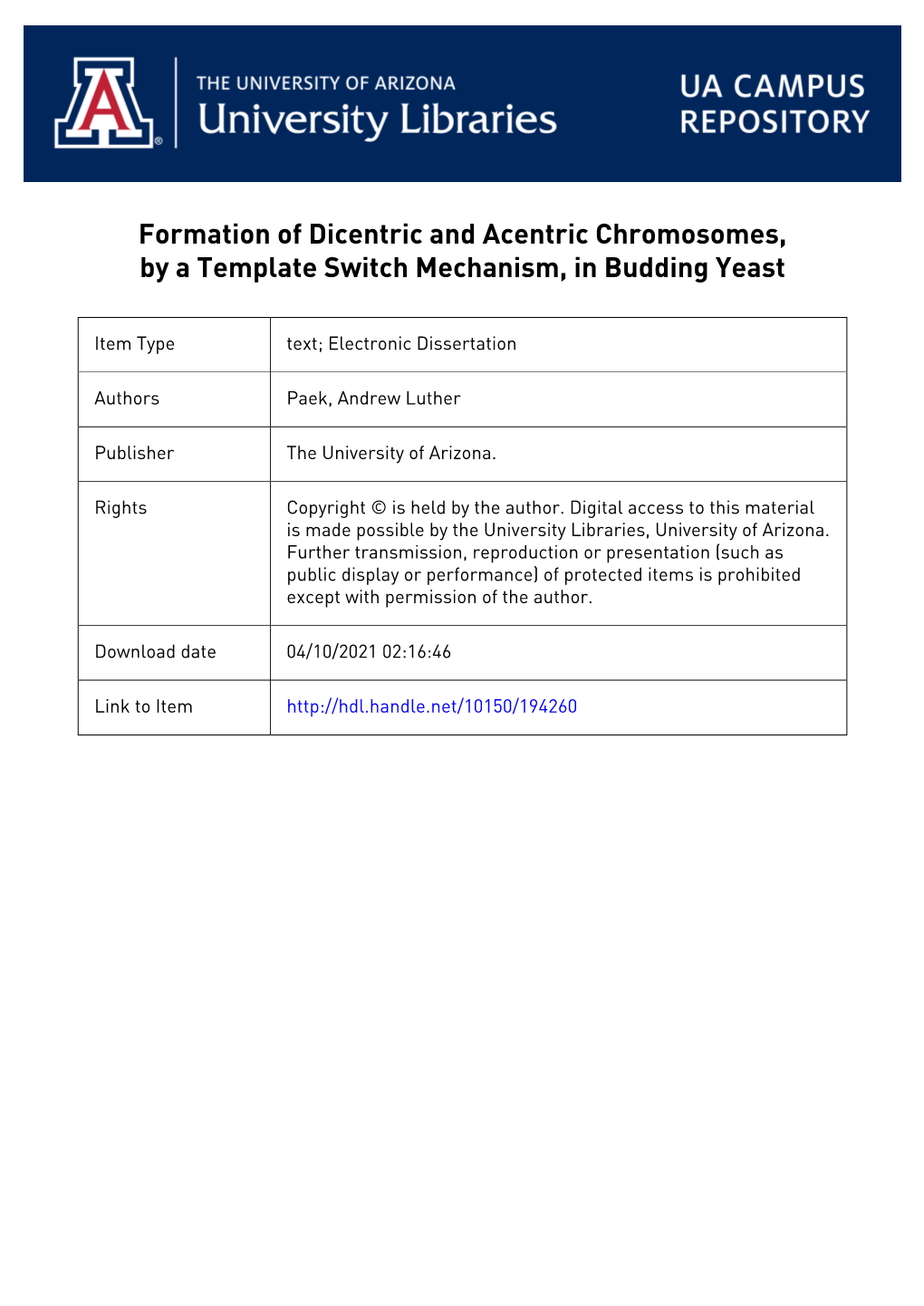
Load more
Recommended publications
-
Epigenetic Control of Mammalian Centromere Protein Binding: Does DNA Methylation Have a Role?
Journal of Cell Science 109, 2199-2206 (1996) 2199 Printed in Great Britain © The Company of Biologists Limited 1996 JCS3386 Epigenetic control of mammalian centromere protein binding: does DNA methylation have a role? Arthur R. Mitchell*, Peter Jeppesen, Linda Nicol†, Harris Morrison and David Kipling MRC Human Genetics Unit, Western General Hospital, Crewe Road, Edinburgh EH4 2XU, UK *Author for correspondence (internet [email protected]) †Present address: MRC Reproductive Biology Unit, Edinburgh, UK SUMMARY Chromosome 1 of the inbred mouse strain DBA/2 has a block of minor satellite DNA sequences on chromosome 1. polymorphism associated with the minor satellite DNA at The binding of the CENP-E protein does not appear to be its centromere. The more terminal block of satellite DNA affected by demethylation of the minor satellite sequences. sequences on this chromosome acts as the centromere as We present a model to explain these observations. This shown by the binding of CREST ACA serum, anti-CENP- model may also indicate the mechanism by which the B and anti-CENP-E polyclonal sera. Demethylation of the CENP-B protein recognises specific sites within the arrays minor satellite DNA sequences accomplished by growing of minor satellite DNA on mouse chromosomes. cells in the presence of the drug 5-aza-2′-deoxycytidine results in a redistribution of the CENP-B protein. This protein now binds to an enlarged area on the more terminal Key words: Centromere satellite DNA, Demethylation, Centromere block and in addition it now binds to the more internal antibody INTRODUCTION A common feature of many mammalian pericentromeric domains is that they contain families of repetitive DNA The centromere of mammalian chromosomes is recognised at sequences (Singer, 1982). -
Chromosomal Rearrangements Genetic Variation Alterationsalterations Inin Chromosomechromosome Structurestructure
chromosomal rearrangements Genetic variation AlterationsAlterations inin ChromosomeChromosome StructureStructure ! There are two primary ways in which the structure of chromosomes can be altered – 1. The total amount of genetic information in the chromosome can change " Decrease: Deficiencies/Deletions " Increase: Duplications & Insertions – 2. The genetic material may remain the same, but is rearranged " Inversions " Translocations PeCtoerp Jy.r Riguhsts e©llT, ihGee nMetciGcsr: aCwop-Hyriilgl hCt o©m Ppeaanriseosn, IEndcu.c Pateiromn,i sInsico.,n p ruebqliusihriendg faosr B reenpjarmodinu cCtuiomnm oirn gdsisplay 3 Chromosomal aberations/ rearrangements Chromosomal abberations/ rearrangements deletion Duplication Inversion translocation. Chromosomal abberations/ rearrangements • For chromosomal rearrangement to occur, there has to be two or more double-stranded breaks in the chromosomes of a cell. • DSBs are potentially lethal, unless they are repaired by repair enzymes. Chromosomal rearrangements • If the two ends of the same break are rejoined, the original DNA order is restored. • If the ends of two different breaks are joined together, results in a chromosomal rearrangement. • The only chromosomal rearrangements that survive meiosis are those that produce DNA molecules that have one centromere and two telomeres. • acentric chromosome: Without a centromere • Do not get dragged to either pole at anaphase of mitosis or meiosis Chromosomal • Are not incorporated into either progeny nucleus. rearrangements Therefore acentric chromosomes are not inherited. Chromosomal Re-arragements • Dicentric chromosome: With two centromere • pulled simultaneously to opposite poles at anaphase, forming an anaphase bridge. • Generally do not get incorporated into either progeny cell. • A chromosome lacking a telomere, cannot replicate properly Chromosomal • The larger the segment Re-arragements that is lost or duplicated, the more chance, that it will cause phenotypic abnormalities. -
Nonrandom Distribution of Interhomolog Recombination Events Induced by Breakage of a Dicentric Chromosome in Saccharomyces Cerevisiae
INVESTIGATION Nonrandom Distribution of Interhomolog Recombination Events Induced by Breakage of a Dicentric Chromosome in Saccharomyces cerevisiae Wei Song,* Malgorzata Gawel,* Margaret Dominska,* Patricia W. Greenwell,* Einat Hazkani-Covo,* Kerry Bloom,† and Thomas D. Petes*,1 *Department of Molecular Genetics and Microbiology, Duke University Medical Center, Durham, North Carolina 27710, and †Department of Biology, University of North Carolina, Chapel Hill, North Carolina 27599 ABSTRACT Dicentric chromosomes undergo breakage in mitosis, resulting in chromosome deletions, duplications, and translocations. In this study, we map chromosome break sites of dicentrics in Saccharomyces cerevisiae by a mitotic recombination assay. The assay uses a diploid strain in which one homolog has a conditional centromere in addition to a wild-type centromere, and the other homolog has only the wild-type centromere; the conditional centromere is inactive when cells are grown in galactose and is activated when the cells are switched to glucose. In addition, the two homologs are distinguishable by multiple single-nucleotide polymorphisms (SNPs). Under conditions in which the conditional centromere is activated, the functionally dicentric chromosome undergoes double-stranded DNA breaks (DSBs) that can be repaired by mitotic recombination with the homolog. Such recombination events often lead to loss of heterozygosity (LOH) of SNPs that are centromere distal to the crossover. Using a PCR-based assay, we determined the position of LOH in multiple independent recombination events to a resolution of 4 kb. This analysis shows that dicentric chromosomes have re- combination breakpoints that are broadly distributed between the two centromeres, although there is a clustering of breakpoints within 10 kb of the conditional centromere. -
Expandable and Reversible Copy Number Amplification Drives Rapid Adaptation to Antifungal Drugs Robert T Todd, Anna Selmecki*
RESEARCH ADVANCE Expandable and reversible copy number amplification drives rapid adaptation to antifungal drugs Robert T Todd, Anna Selmecki* Department of Microbiology and Immunology, University of Minnesota Medical School, Minneapolis, Minnesota, United States Abstract Previously, we identified long repeat sequences that are frequently associated with genome rearrangements, including copy number variation (CNV), in many diverse isolates of the human fungal pathogen Candida albicans (Todd et al., 2019). Here, we describe the rapid acquisition of novel, high copy number CNVs during adaptation to azole antifungal drugs. Single- cell karyotype analysis indicates that these CNVs appear to arise via a dicentric chromosome intermediate and breakage-fusion-bridge cycles that are repaired using multiple distinct long inverted repeat sequences. Subsequent removal of the antifungal drug can lead to a dramatic loss of the CNV and reversion to the progenitor genotype and drug susceptibility phenotype. These findings support a novel mechanism for the rapid acquisition of antifungal drug resistance and provide genomic evidence for the heterogeneity frequently observed in clinical settings. Introduction The evolution of antifungal drug resistance is an urgent threat to human health worldwide, particu- larly for hospitalized and immune-compromised individuals (Perea and Patterson, 2002; Pfal- ler, 2012; Vandeputte et al., 2012). Only three classes of antifungal drugs are currently available *For correspondence: [email protected] and resistance to all three classes occurred for the first time in the emerging fungal pathogen Can- dida auris (Chen and Sorrell, 2007; Ghannoum and Rice, 1999; Lockhart et al., 2017). Importantly, Competing interests: The the mechanisms and dynamics of acquired antifungal drug resistance, in vitro or in a patient under- authors declare that no going antifungal drug therapy, are not fully understood. -
Cytokinesis Breaks Dicentric Chromosomes Preferentially at Pericentromeric Regions and Telomere Fusions
Downloaded from genesdev.cshlp.org on October 2, 2021 - Published by Cold Spring Harbor Laboratory Press Cytokinesis breaks dicentric chromosomes preferentially at pericentromeric regions and telomere fusions Virginia Lopez,1,2,5 Natalja Barinova,1,2,5 Masayuki Onishi,3 Sabrina Pobiega,1,2 John R. Pringle,3 Karine Dubrana,2,4 and Stephane Marcand1,2 1Laboratoire Telom eres et Reparation du Chromosome, Service InstabiliteG en etique Reparation et Recombinaison, Institut de Radiobiologie Moleculaire et Cellulaire, Commissariat a l’Energie Atomique et aux Energies Alternatives, 92265 Fontenay-aux- Roses, France; 2UMR967, Institut National de la Sante et de la Recherche Medicale, 92265 Fontenay-aux-Roses, France; 3Department of Genetics, Stanford University School of Medicine, Stanford, California 94305, USA; 4Laboratoire Instabilite Gen etique et Organisation Nucleaire, Service InstabiliteG en etique Reparation et Recombinaison, Institut de Radiobiologie Moleculaire et Cellulaire, Commissariat a l’Energie Atomique et aux Energies Alternatives, 92265 Fontenay-aux-Roses, France Dicentric chromosomes are unstable products of erroneous DNA repair events that can lead to further genome rearrangements and extended gene copy number variations. During mitosis, they form anaphase bridges, resulting in chromosome breakage by an unknown mechanism. In budding yeast, dicentrics generated by telomere fusion break at the fusion, a process that restores the parental karyotype and protects cells from rare accidental telomere fusion. Here, we observed that dicentrics lacking telomere fusion preferentially break within a 25- to 30-kb-long region next to the centromeres. In all cases, dicentric breakage requires anaphase exit, ruling out stretching by the elongated mitotic spindle as the cause of breakage. Instead, breakage requires cytokinesis. -
Diatom Centromeres Suggest a Mechanism for Nuclear DNA Acquisition
Diatom centromeres suggest a mechanism for nuclear PNAS PLUS DNA acquisition Rachel E. Dinera,b, Chari M. Noddingsc, Nathan C. Lianc, Anthony K. Kangc, Jeffrey B. McQuaida,b, Jelena Jablanovicb, Josh L. Espinozab, Ngocquynh A. Nguyenc, Miguel A. Anzelmatti Jr.b, Jakob Janssonc, Vincent A. Bielinskic, Bogumil J. Karasc,1, Christopher L. Dupontb, Andrew E. Allena,b, and Philip D. Weymanc,2 aIntegrative Oceanography Division, Scripps Institution of Oceanography, University of California, San Diego, La Jolla, CA 92037; bMicrobial and Environmental Genomics Group, J. Craig Venter Institute, La Jolla, CA 92037; and cSynthetic Biology and Bioenergy Group, J. Craig Venter Institute, La Jolla, CA 92037 Edited by James A. Birchler, Division of Biological Sciences, University of Missouri, Columbia, MO, and approved June 13, 2017 (received for review January 17, 2017) Centromeres are essential for cell division and growth in all eukary- transposons, which can vary substantially in copy number and otes, and knowledge of their sequence and structure guides the organization (16). A common feature of centromeric DNA in many development of artificial chromosomes for functional cellular biol- eukaryotes is low-GC content. Centromeres of Schizosaccharomyces ogy studies. Centromeric proteins are conserved among eukaryotes; pombe and other yeast species feature an unconserved core of AT- however, centromeric DNA sequences are highly variable. We rich DNA sequence often surrounded by inverted repeats (17–20). combined forward and reverse genetic approaches with chromatin The centromeres of the protist Plasmodium have no apparent se- immunoprecipitation to identify centromeres of the model diatom quence similarity besides being 2–4-kb regions of extremely low-GC Phaeodactylum tricornutum . -
Dicentric X Isochromosomes in Man R
J Med Genet: first published as 10.1136/jmg.13.6.496 on 1 December 1976. Downloaded from Journal of Medical Genetics (1976). 13, 496-500. Dicentric X isochromosomes in man R. T. HOWELL, S. H. ROBERTS, and R. J. BEARD From the Child Health Laboratories, Department of Child Health, University Hospital of Wales, Heath Park, Cardiff Summary. Four cases of Turner's syndrome are presented in which an appa- rent X isochromosome i(Xq) has been found to possess two regions of centromeric heterochromatin. It is suggested that these chromosomes were isodicentric struc- tures capable offunctioning as monocentric elements as a result ofthe inactivation of one centromere. The prevalence of mosaicism is believed to be a consequence of the dicentric nature ofthese chromosomes, and it is considered possible that a high proportion of X isochromosomes are structurally dicentric. Banding patterns showed that the exchange site involved in the formation ofthe dicentric chromosome was different in at least three ofthe cases. The possibility that certain X isochromosomes Methods were dicentric was first postulated by de la Chapelle Chromosome preparations were made from peripheral et al (1966). In 2 of 5 cases investigated they noted blood lymphocyte cultures ofall 4 patients using standard that the isochromosome had an apparently elon- procedures. Preparations from skin fibroblast cultures an gated centromeric region. In 3 cases they found (Cases 1 and 2) and ovarian tissue culture (Case 3)copyright. abnormal anaphase configurations such as bridges, were also investigated. Slides were either stained with pseudochiasmata, and lagging chromosomes, indica- aceto-orcein in order to examine chromosome morpho- tive of the presence of a dicentric chromosome. -
Human Dicentric Y Chromosomes Case Report and Review of the Literature MAIMON M
J Med Genet: first published as 10.1136/jmg.10.1.74 on 1 March 1973. Downloaded from Journal of Medical Genetics (1973). 10, 74. Human Dicentric Y Chromosomes Case Report and Review of the Literature MAIMON M. COHEN,* MARGARET H. MAcGILLIVRAY, VINCENT J. CAPRARO, and THOMAS A. ACETO Divisions of Human Genetics and Endocrinology, Department of Pediatrics, State University of New York at Buffalo School of Medicine and Children's Hospital of Buffalo, Buffalo, New York, USA Summary. A phenotypic female with histological evidence of mixed gonadal dysgenesis, and 45,X/46,X,dic(Yq) mosaicism is described. A review of the litera- ture yielded 15 additional cases of dicentric Y chromosomes. Among the cases, a wide range ofvariation in phenotype, external genitalia, histology, and chromosomal findings was observed. Factors possibly contributing to such variability are dis- cussed and include: the exact site of breakage and exchange in the Y chromosome; the timing of dicentric formation (meiotic vs mitotic); the occurrence of non-dis- junction; and the presence or absence of a Y chromosome in cells of the gonadal anlage during a critical ontogenic period. Morphologically the human Y chromosome is, logical and cytogenetic variability. The possible probably, the most variable chromosome of the bases for such disparity are considered in this paper. human karyotype. Extreme variations of length have been observed both in normal individuals and in individuals with physical abnormalities (Makino Subjects and Methods et al, 1963; Gripenberg, 1964; Cohen, Shaw, and Case Report. L.P., an 11-year-old girl, was the MacCluer, 1966; McKenzie et al, 1972). -
MINI-REVIEW Centric and Pericentric Chromosome Rearrangements In
Leukemia (1999) 13, 671–678 1999 Stockton Press All rights reserved 0887-6924/99 $12.00 http://www.stockton-press.co.uk/leu MINI-REVIEW Centric and pericentric chromosome rearrangements in hematopoietic malignancies R Berger and M Busson-Le Coniat INSERM U434 and CNRS SD 401 No. 434, Institut de Ge´ne´tique Mole´culaire, Paris, France Cytogenetic and fluorescence in situ hybridization (FISH) lymphoblastic leukemia, have been described as nonrandom analysis of 10 patients with various hematopoietic malig- abnormalities in hematopoietic malignancies.6–10 Other nancies revealed the presence of dicentric chromosomes or pericentric chromosome rearrangements. Dicentrics were only examples of dicentric chromosomes implying various chro- ascertained by FISH studies in six patients. Two types of peri- mosomes occurring as clonal abnormalities, have been centric chromosome rearrangements have been observed: reported to be present in hematopoietic disorders (Table 1). ‘classical’ dicentrics with two clearly separated centromeric Dicentrics may be difficult to detect with banding techniques regions, and more unusual rearrangements with a breakpoint if the two centromeres are very closely located on rearranged within the centromeric or heterochromatic area, but outside the chromosomes. This difficulty can be overcome by use of FISH, alphoid domain. FISH analysis of partial chromosome 1 q dupli- cations present in three Burkitt lymphoma cell lines confirmed and it has been shown that a large number of isochromosomes the partial involvement of the non-alphoid centromeric domain identified by chromosome banding analysis actually are iso- in the duplicated chromosome segment. The incidence of cen- dicentric chromosomes (Table 1). The overall frequency of tromeric and pericentromeric rearrangements in hematopoietic isochromosomes in human malignancies was 9.9% in 18 160 malignancies may be higher than hitherto admitted. -
LECTURE 9: CHROMOSOMAL REARRANGEMENTS II Reading for This and Last Lecture: Ch
Amacher Lecture 9 (9/22/08) LECTURE 9: CHROMOSOMAL REARRANGEMENTS II Reading for this and last lecture: Ch. 14, p. 489-515; Fig. 7.9 (p 215) & associated text; optional reading in the Drosophila portrait (skim section D.3) Problems for this and last lecture: Ch. 14, solved probs I, II; also 6, 8, 11-14, 17, 20, 21, 24 Announcements: *Are you doing the problems? Doing the problems is by far the best way to study for the class. *No office hours this week. Next week, office hours are Thursday 10/2 1:30-3:30 and Friday 10/3 from 10-noon. *This week, beginning September 24th, Section 114 (Wed 2-3 pm) moves from 2032 VLSB to 2038 VLSB Today we'll continue talking about rearrangements, focusing on inversions and translocations. Inversion: A rearrangement in which a chromosomal segment is rotated 180 degrees. The symbol used is In. Inversions in which the rotated segment includes the centromere are called pericentric inversions; those in which the rotated segment is located completely on one chromosomal arm and do not include the centromere are called paracentric inversions. Inversions can occur when two double-strand breaks release a chromosomal region that inverts before religating to flanking DNA, or by intrachromosomal recombination. Pericentric (includes centromere; "peri" means "around, about") ABC - cen - DEFGH AD - cen - CBEFGH Paracentric (does not include centromere; "para" means "beside, beyond") cen - ABCDEFGH cen - ADCBEFGH Even though gene order is changed in an inversion, many inversions do not cause abnormal phenotypes. Many inversions can be made homozgous, and inversions can be detected in haploid organisms. -
Stabilization of Dicentric Chromosomes in Saccharomyces Cerevisiae by Telomere Addition to Broken Ends Or by Centromere Deletion
The EMBO Journal vol.8 no. 1 pp.247 - 254, 1989 Stabilization of dicentric chromosomes in Saccharomyces cerevisiae by telomere addition to broken ends or by centromere deletion Daniel Jager and Peter Philippsen1 Molecular studies with respect to the stabilization processes of dicentric chromosomes have so far only been performed Department of Microbiology, Biozentrum, University of Basel, cerevisiae. This simple Klingelbergstrasse 70, CH-4056 Basel, Switzerland, and 'Institute of with the yeast Saccharomyces Microbiology and Molecular Biology, Justus Liebig University eucaryote has several advantages for such studies. It is Giessen, Frankf urterstrasse 107, D-6300 Giessen, FRG genetically well characterized, tolerates extensive Communicated by P.Philippsen aneuploidy, can easily be transformed with recombinant DNA and it is the only organism for which cloned centro- We introduced CEN6 DNA via integrative transformation mere DNA (CEN DNA) is available, thus allowing con- into the right arm of chromosome H in a haploid Sac- structions of dicentric DNA molecules with predetermined charomyces cerevisiae strain thus creating a dicentric sequence arrangements. chromosome. The majority of the transformed cells did Three different experimental approaches have been used not grow into colonies as concluded from control trans- to construct dicentric chromosomes. In the first approach formations with mutated CEN6 DNA. Five percent of the identical or non-identical CEN DNAs were cloned into initial transformants with the wild-type centromere gave plasmids capable of autonomous replication in S. cerevisiae rise to well growing cells. We analysed the probable fate (Mann and Davis, 1983; Oertel and Mayer, 1984; Koshland of the dicentric chromosome in two transformants by et al., 1987). -
Variants and Health
Help Me Understand Genetics Variants and Health Reprinted from MedlinePlus Genetics U.S. National Library of Medicine National Institutes of Health Department of Health & Human Services Table of Contents 1 What is a gene variant and how do variants occur? 1 2 How can gene variants affect health and development? 3 3 Do all gene variants affect health and development? 4 4 What kinds of gene variants are possible? 6 5 Can a change in the number of genes affect health and development? 12 6 Can changes in the number of chromosomes affect health and development? 13 7 Can changes in the structure of chromosomes affect health and development? 19 8 Can changes in noncoding DNA affect health and development? 26 9 Can changes in mitochondrial DNA affect health and development? 28 10 What are complex or multifactorial disorders? 29 11 What does it mean to have a genetic predisposition to a disease? 30 12 How are gene variants involved in evolution? 32 13 What information can statistics provide about a genetic condition? 34 Reprinted from MedlinePlus Genetics (https://medlineplus.gov/genetics/) i 14 How are genetic conditions and genes named? 36 Reprinted from MedlinePlus Genetics (https://medlineplus.gov/genetics/) ii Variants and Health 1 What is a gene variant and how do variants occur? A gene variant is a permanent change in the DNA sequence that makes up a gene. This type of genetic change used to be known as a gene mutation, but because changes in DNA do not always cause disease, it is thought that gene variant is a more accurate term.