NPM-ALK: a Driver of Lymphoma Pathogenesis and a Therapeutic Target
Total Page:16
File Type:pdf, Size:1020Kb
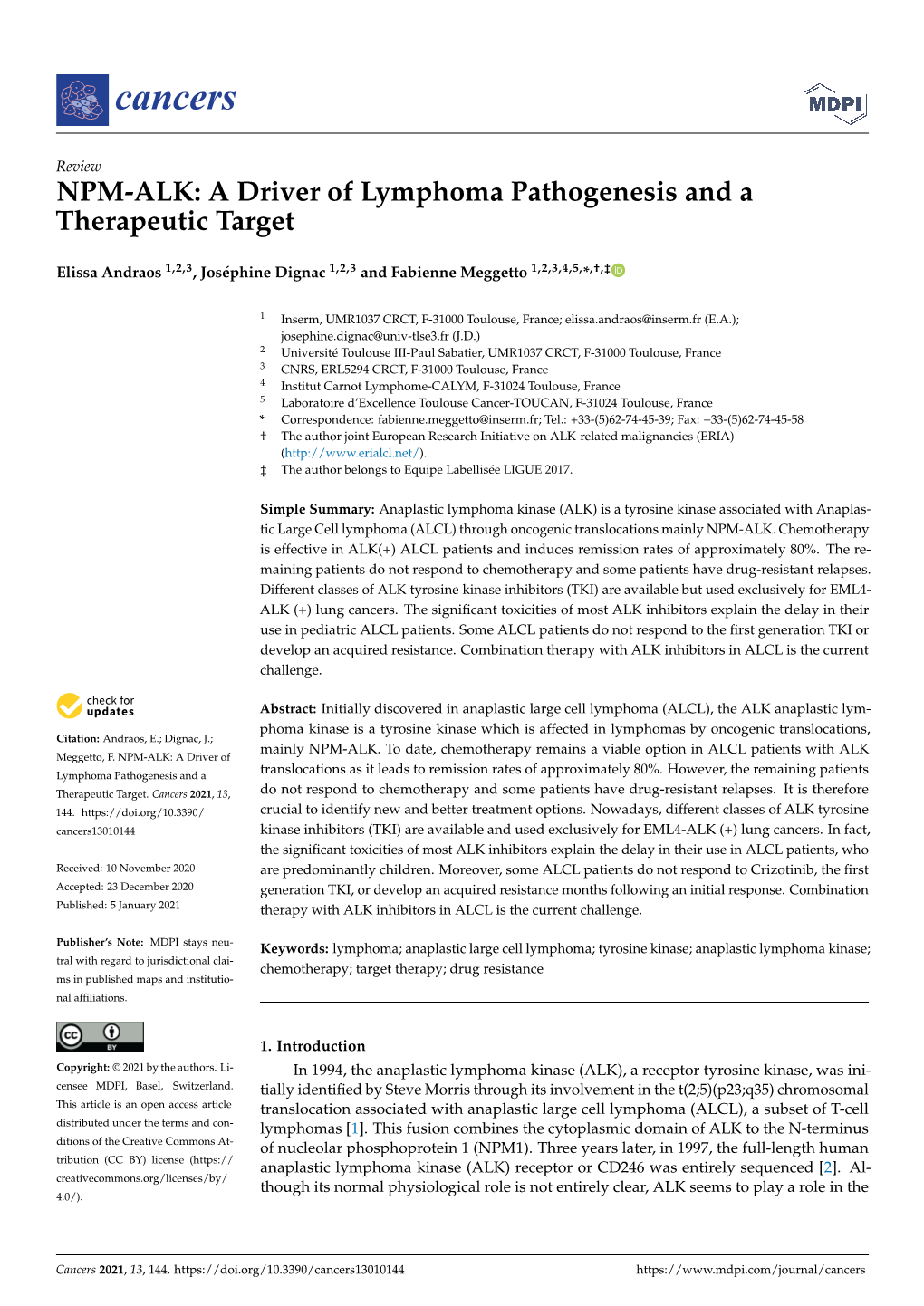
Load more
Recommended publications
-
DNA Damage and the Activation of the P53 Pathway Mediate Alterations in Metabolic and Secretory Functions of Adipocytes
3062 Diabetes Volume 65, October 2016 Bastien Vergoni,1,2 Pierre-Jean Cornejo,1,2 Jérôme Gilleron,1,2 Mansour Djedaini,1,2 Franck Ceppo,1,2 Arnaud Jacquel,2,3 Gwennaelle Bouget,1,2 Clémence Ginet,1,2 Teresa Gonzalez,1,2,4 Julie Maillet,5,6,7 Véronique Dhennin,5,6,7 Marie Verbanck,5,6,7 Patrick Auberger,2,3 Philippe Froguel,5,6,7,8 Jean-François Tanti,1,2 and Mireille Cormont1,2 DNA Damage and the Activation of the p53 Pathway Mediate Alterations in Metabolic and Secretory Functions of Adipocytes Diabetes 2016;65:3062–3074 | DOI: 10.2337/db16-0014 Activation of the p53 pathway in adipose tissue contributes Insulin resistance is associated with obesity and is a major to insulin resistance associated with obesity. However, the risk factor for type 2 diabetes. Adipose tissue (AT) plays mechanisms of p53 activation and the effect on adipocyte an important role in glucose and lipid homeostasis (1,2), functions are still elusive. Here we found a higher level of and AT dysfunction associated with obesity has emerged DNA oxidation and a reduction in telomere length in adipose as a critical event for the development of insulin resis- tissueofmicefedahigh-fatdietandanincreaseinDNA tance. Low-grade inflammation and oxidative and hypoxic damage and activation of the p53 pathway in adipocytes. stresses both develop in obese AT and are involved in the Interestingly, hallmarks of chronic DNA damage are visible alteration of adipocyte functions (3–6). Therefore, activa- at the onset of obesity. Furthermore, injection of lean mice tion of stress-signaling pathways in adipocytes is critically with doxorubicin, a DNA damage-inducing drug, increased involved in AT dysfunction and insulin resistance (7–10). -
Parameter IV Dose (1 Mg/Kg) Oral Dose (10 Mg/Kg)
AG-221, a First-in-Class Therapy Targeting Acute Myeloid Leukemia Harboring Oncogenic IDH2 Mutations Katharine Yen, Jeremy Travins, Fang Wang, Muriel D. David, Erin Artin, Kimberly Straley, Anil Padyana, Stefan Gross, Byron DeLaBarre, Erica Tobin, Yue Chen, Raj Nagaraja, Sung Choe, Lei Jin, Zenon Konteatis, Giovanni Cianchetta, Jeffrey O. Saunders, Francesco G. Salituro, Cyril Quivoron, Paule Opolon, Olivia Bawa, Véronique Saada, Angélo Paci, Sophie Broutin, Olivier Bernard, Stéphane de Botton, Benoît S. Marteyn, Monika Pilichowska, YingXia Xu, Cheng Fang, Fan Jiang, Wentao Wei, Shengfang Jin, Lee Silverman, Wei Liu, Hua Yang, Lenny Dang, Marion Dorsch, Virginie Penard-Lacronique, Scott A. Biller, Shin-San Michael Su SUPPLEMENTARY TABLES Supplementary Table 1. Drug metabolism and pharmacokinetic attributes of AG-221 Parameter IV dose (1 mg/kg) Oral dose (10 mg/kg) CL (l/h/kg) 0.83 ± 0.10 NA Vss (l/kg) 5.5 ± 1.6 NA AUC (h.ng/ml) 1,200 ± 130 5,000 ± 610 t1/2 (h) 5.4 ± 1.8 5.4 ± 1.2 F (%) NA 41 ± 5.0 IV and oral pharmacokinetic parameters of AG-221 in male Sprague-Dawley rats (mean ± s.d., n = 3). AUC, area under plasma concentration curve; CL, clearance; F, absolute oral bioavailability; IV, intravenous; NA, not applicable; t1/2, terminal half-life; Vss, volume of distribution at steady state. Page 1 of 12 Supplementary Table 2. Selectivity of AG-221 confirmed by testing against a panel of kinases Enzyme IC50 of AG-221 IC50 of reference Reference (nM) (nM) P13K >10,000 9.1 PI 103 JNK2 >10,000 5741.2 Staurosporine AKT1 >10,000 9.8 Staurosporine -
Moyamoya Disease Susceptibility Gene RNF213 Links Inflammatory
www.nature.com/scientificreports OPEN Moyamoya disease susceptibility gene RNF213 links inflammatory and angiogenic signals in Received: 19 March 2015 Accepted: 03 July 2015 endothelial cells Published: 17 August 2015 Kazuhiro Ohkubo1,*, Yasunari Sakai1,*, Hirosuke Inoue1, Satoshi Akamine1, Yoshito Ishizaki1, Yuki Matsushita1, Masafumi Sanefuji1, Hiroyuki Torisu1,3, Kenji Ihara1,2, Marco Sardiello4 & Toshiro Hara1 Moyamoya disease (MMD) is a cerebrovascular disorder characterized by occlusive lesions of the circle of Willis. To date, both environmental and genetic factors have been implicated for pathogenesis of MMD. Allelic variations in RNF213 are known to confer the risk of MMD; however, functional roles of RNF213 remain to be largely elusive. We herein report that pro-inflammatory cytokines, IFNG and TNFA, synergistically activated transcription of RNF213 both in vitro and in vivo. Using various chemical inhibitors, we found that AKT and PKR pathways contributed to the transcriptional activation of RNF213. Transcriptome-wide analysis and subsequent validation with quantitative PCR supported that endogenous expression of cell cycle-promoting genes were significantly decreased with knockdown of RNF213 in cultured endothelial cells. Consistently, these cells showed less proliferative and less angiogenic profiles. Chemical inhibitors for AKT (LY294002) and PKR (C16) disrupted their angiogenic potentials, suggesting that RNF213 and its upstream pathways cooperatively organize the process of angiogenesis. Furthermore, RNF213 down-regulated expressions of matrix metalloproteases in endothelial cells, but not in fibroblasts or other cell types. Altogether, our data illustrate that RNF213 plays unique roles in endothelial cells for proper gene expressions in response to inflammatory signals from environments. Moyamoya disease (MMD) represents a specific intracranial vascular disorder characterized by progres- sive, occlusive lesions of internal carotid arteries and branches in the circle of Willis1,2. -
Selective Insulin Signaling Through a and B Insulin Receptors Regulates Transcription of Insulin and Glucokinase Genes in Pancreatic  Cells
Molecular Cell, Vol. 7, 559±570, March, 2001, Copyright 2001 by Cell Press Selective Insulin Signaling through A and B Insulin Receptors Regulates Transcription of Insulin and Glucokinase Genes in Pancreatic  Cells Barbara Leibiger,*§ Ingo B. Leibiger,*§k ceptors as the primary target, include signaling via mito- Tilo Moede,* Sabine Kemper,* gen-activated protein (MAP) kinases and phosphoinosi- Rohit N. Kulkarni,² C. Ronald Kahn,² tol-3 kinase (PI3K). The insulin receptor (IR), the first Lina Moitoso de Vargas,³ and Per-Olof Berggren* step in these cascades, exists in two isoforms as a result *The Rolf Luft Center for Diabetes Research of alternative mRNA splicing of the 11th exon of the insulin Department of Molecular Medicine proreceptor transcript (Seino et al., 1989). The A type Karolinska Institutet (IR-A), or Ex11Ϫ (Ullrich et al., 1985), lacks whereas the S-171 76 Stockholm B type (IR-B), or Ex11ϩ (Ebina et al., 1985), contains Sweden the respective sequence coding for 12 amino acids in ² Research Division the C terminus of the ␣ chain of the receptor. To date, Joslin Diabetes Center and no insulin-induced effect has been reported that dis- Department of Medicine criminates signaling via A- and B-type receptors. In fact, Harvard Medical School the functional significance of these IR isoforms remains Boston, Massachusetts 02215 unclear. ³ Department of Medicine Recent studies have shown that the insulin-producing New England Medical Center and pancreatic  cell is a target for insulin action, with insulin Tufts University School of Medicine effects on transcription, translation, Ca2ϩ flux, and exo- Boston, Massachusetts 02111 cytosis (Leibiger et al., 1998a, 2000; Xu and Rothenberg, 1998; Xu et al., 1998; Aspinwall et al., 1999; Kulkarni et al., 1999a). -
NPM1-ALK (Human) Recombinant Gene Summary: the 2;5 Chromosomal Translocation Is Protein Frequently Associated with Anaplastic Large Cell Lymphomas (Alcls)
NPM1-ALK (Human) Recombinant Gene Summary: The 2;5 chromosomal translocation is Protein frequently associated with anaplastic large cell lymphomas (ALCLs). The translocation creates a fusion Catalog Number: P5782 gene consisting of the ALK (anaplastic lymphoma kinase) gene and the nucleophosmin (NPM) gene: the 3' Regulation Status: For research use only (RUO) half of ALK, derived from chromosome 2, is fused to the 5' portion of NPM from chromosome 5. A recent study Product Description: Human NPM1-ALK (BAA08343.1, shows that the product of the NPM-ALK fusion gene is 1 a.a. - 680 a.a.) partial recombinant protein with GST oncogenic. The deduced amino acid sequences reveal tag expressed in Baculovirus infected Sf21 cells. that ALK is a novel receptor protein-tyrosine kinase having a putative transmembrane domain and an Host: Insect extracellular domain. These sequences are absent in the product of the transforming NPM-ALK gene. ALK shows Theoretical MW (kDa): 103 the greatest sequence similarity to LTK (leukocyte tyrosine kinase). ALK plays an important role in the Applications: Func, SDS-PAGE development of the brain and exerts its effects on (See our web site product page for detailed applications specific neurons in the nervous system. [provided by information) RefSeq] Protocols: See our web site at http://www.abnova.com/support/protocols.asp or product page for detailed protocols Form: Liquid Preparation Method: Baculovirus infected insect cell (Sf21) expression system Purification: Glutathione sepharose chromatography Purity: 81 % by SDS-PAGE/CBB staining Activity: The activity was measured by off-chip mobility shift assay. The enzyme was incubated with fluorescence-labeled substrate and Mg (or Mn)/ATP. -
Insulin–Insr Signaling Drives Multipotent Progenitor Differentiation Toward Lymphoid Lineages
Article Insulin–InsR signaling drives multipotent progenitor differentiation toward lymphoid lineages Pengyan Xia,1* Shuo Wang,1* Ying Du,1 Guanling Huang,1,2 Takashi Satoh,3 Shizuo Akira,3 and Zusen Fan1 1Key Laboratory of Infection and Immunity of CAS, Institute of Biophysics, Chinese Academy of Sciences, Beijing 100101, China 2University of Chinese Academy of Sciences, Beijing 100049, China 3Department of Host Defense, Research Institute for Microbial Diseases (RIMD), Osaka University, Suita, Osaka 565-0871, Japan The lineage commitment of HSCs generates balanced myeloid and lymphoid populations in hematopoiesis. However, the un- derlying mechanisms that control this process remain largely unknown. Here, we show that insulin–insulin receptor (InsR) signaling is required for lineage commitment of multipotent progenitors (MPPs). Deletion of Insr in murine bone marrow causes skewed differentiation of MPPs to myeloid cells. mTOR acts as a downstream effector that modulates MPP differentiation. mTOR activates Stat3 by phosphorylation at serine 727 under insulin stimulation, which binds to the promoter of Ikaros, lead- ing to its transcription priming. Our findings reveal that the insulin–InsR signaling drives MPP differentiation into lymphoid lineages in early lymphopoiesis, which is essential for maintaining a balanced immune system for an individual organism. Hematopoiesis is the process of producing all compo- Insulin, as the primary anabolic hormone, modulates a nents of the blood system from hematopoietic stem cells variety of physiological processes, including growth, differ- (HSCs; Naik et al., 2013; Mendelson and Frenette, 2014; entiation, apoptosis, and synthesis and breakdown of lipid, Walter et al., 2015). HSCs are quiescent, self-renewable pro- protein, and glucose (Samuel and Shulman, 2012). -
PSM, a Mediator of PDGF-BB-, IGF-I-, and Insulin-Stimulated Mitogenesis
Oncogene (2000) 19, 39 ± 50 ã 2000 Macmillan Publishers Ltd All rights reserved 0950 ± 9232/00 $15.00 www.nature.com/onc PSM, a mediator of PDGF-BB-, IGF-I-, and insulin-stimulated mitogenesis Heimo Riedel*,1,2, Nasim Yousaf1, Yuyuan Zhao4, Heping Dai1,3, Youping Deng1 and Jian Wang1 1Department of Biological Sciences, Wayne State University, Detroit, Michigan, MI 48202, USA; 2Member, Barbara Ann Karmanos Cancer Institute, Detroit, Michigan, MI 48201, USA PSM/SH2-B has been described as a cellular partner of Introduction the FceRI receptor, insulin receptor (IR), insulin-like growth factor-I (IGF-I) receptor (IGF-IR), and nerve The platelet-derived growth factor (PDGF) family growth factor receptor (TrkA). A function has been represents key mitogenic and chemotactic factors that proposed in neuronal dierentiation and development but have been exploited by the Simian sarcoma virus v-sis its role in other signaling pathways is still unclear. To oncogene which results in altered PDGF expression further elucidate the physiologic role of PSM we have (Water®eld et al., 1983). Normal cellular PDGF identi®ed additional mitogenic receptor tyrosine kinases appears in three distinct isoforms as any combination as putative PSM partners including platelet-derived of the PDGF A and B chains which act in paracrine growth factor (PDGF) receptor (PDGFR) beta, hepato- and autocrine mechanisms (Heldin, 1993). PDGF cyte growth factor receptor (Met), and ®broblast growth receptor (PDGFR) signal transduction appears to be factor receptor. We have mapped Y740 as a site of a largely membrane delineated event (Hughes et al., PDGFR beta that is involved in the association with 1996). -
Structure of the Tie2 RTK Domain: Self-Inhibition by the Nucleotide Binding Loop, Activation Loop, and C-Terminal Tail
View metadata, citation and similar papers at core.ac.uk brought to you by CORE provided by Elsevier - Publisher Connector Structure, Vol. 8, 1105±1113, November, 2000, 2000 Elsevier Science Ltd. All rights reserved. PII S0969-2126(00)00516-5 Structure of the Tie2 RTK Domain: Self-Inhibition by the Nucleotide Binding Loop, Activation Loop, and C-Terminal Tail Lisa M. Shewchuk,* Anne M. Hassell, Byron Ellis, binding domain and an intracellular kinase domain. Binding of W. D. Holmes, Roderick Davis, Earnest L. Horne, extracellular ligand is believed to promote dimerization, which Sue H. Kadwell, David D. McKee, leads to autophosphorylation and activation of the kinase do- and John T. Moore main [reviewed in 15]. Stringent regulation of the phosphoryla- GlaxoWellcome tion state and activity of the Tie2 kinase domain is crucial to Research Triangle Park, North Carolina 27709 normal vasculature development and maintenance. Tie2 activ- ity is precisely regulated by the opposing actions of agonistic and antagonistic extracellular ligands [16±18]. Tie2 activation Summary requires autophosporylation in response to binding its agonists angiopoietin 1 (Ang1) and Ang4, whereas inactivation occurs Background: Angiogenesis, the formation of new vessels from in response to Ang2 and Ang3. Tie2 mutations, which result the existing vasculature, is a critical process during early devel- in ligand-independent and enhanced autophosphorylation, opment as well as in a number of disease processes. Tie2 (also cause hereditary venous malformations [19, 20]. Conversely, known as Tek) is an endothelium-specific receptor tyrosine transgenic mice that express a kinase-inactive form of Tie2 or kinase involved in both angiogenesis and vasculature mainte- Tie2 null mice die in utero due to defects in their microvascula- nance. -
Increased Expression of NPM1 Suppresses P27kip1 Function in Cancer Cells
cancers Article Increased Expression of NPM1 Suppresses p27Kip1 Function in Cancer Cells Tatsuya Kometani, Takuya Arai and Taku Chibazakura * Department of Bioscience, Tokyo University of Agriculture 1-1-1, Sakuragaoka, Setagaya-ku, Tokyo 156-8502, Japan; [email protected] (T.K.); [email protected] (T.A.) * Correspondence: [email protected] Received: 4 August 2020; Accepted: 5 October 2020; Published: 8 October 2020 Simple Summary: Cancer malignancy frequently correlates with a low expression of p27Kip1, a major cyclin-dependent kinase inhibitor, and the p27 protein level has been reportedly responsible for its antiproliferative function. However, we found the function of overexpressed p27 is suppressed in some cancer cells, suggesting that p27 function is also regulated independently of its protein level. The aim of this study was to clarify this unknown p27 regulatory mechanism and its impact on cancer proliferation. We isolated nucleophosmin isoform 1 (NPM1), which is highly expressed in variety of cancers, as a novel p27-interacting protein. Overexpressing NPM1 in normal cells suppressed and silencing NPM1 in cancer cells rescued the p27 function, respectively, in vitro. Moreover, NPM1 silencing and p27 induction in cancer cells significantly suppressed their proliferation in mouse xenografts. Our findings reveal that NPM1 is a novel p27 functional suppressor and a potential anti-cancer target, especially in cancers with normal p27 expression. Abstract: p27Kip1, a major cyclin-dependent kinase inhibitor, is frequently expressed at low levels in cancers, which correlates with their malignancy. However, in this study, we found a qualitative suppression of p27 overexpressed in some cancer cells. By proteomic screening for factors interacting with p27, we identified nucleophosmin isoform 1 (NPM1) as a novel p27-interacting factor and observed that NPM1 protein was expressed at high levels in some cancer cells. -
Core Transcription Factors, Oct4, Sox2 and Nanog, Individually Form Complexes with Nucleophosmin (Npm1) to Control Embryonic Stem
www.impactaging.com AGING, November 2010, Vol 2 N 11 Research Paper Core transcription factors, Oct4, Sox2 and Nanog, individually form complexes with nucleophosmin (Npm1) to control embryonic stem (ES) cell fate determination Helena Johansson and Stina Simonsson Department of Medical Biochemistry and Cell Biology; Institute of Biomedicine; University of Gothenburg, box 440, 405 30 Gothenburg, Västra Götaland; Sweden Key words: Npm1, Oct4, Sox2, Nanog, ES cells, Tpt1 Received: 10/25/10; accepted: 11/10/10; published on line: 11/12/10 Corresponding author: Stina Simonsson, PhD; E‐mail: [email protected] Copyright: © Johansson and Simonsson. This is an open‐access article distributed under the terms of the Creative Commons A ttribution License, which permits unrestricted use, distribution, and reproduction in any medium, provided the original author and source are credited Abstract: Embryonic stem (ES) cells have therapeutic potential in regenerative medicine, although the molecular mechanism controlling their pluripotency is not completely understood. Depending on interaction partners most proteins can be involved in several different cellular mechanisms. We screened for novel protein‐protein interactions using in situ proximity ligation assays together with specific antibodies directed against known important ES cell proteins. We found that all three core transcription factors, namely Oct4, Sox2 and Nanog, individually formed complexes with nucleophosmin (Npm1). We showed that the Npm1/Sox2 complex was sustained when cells were induced to differentiate by retinoic acid, while decreased in the other differentiation pathways. Moreover, Oct4 also formed individual complexes with translationally controlled tumor protein (Tpt1). Downregulation of Npm1 or Tpt1 increased mRNA levels for genes involved in mesoderm and ectoderm differentiation pathways, respectively, indicative of their involvement in ES cell maintenance. -
Next Generation Gene Sequencing -Solid Tumor
Cancer‐related Mutaon Analysis Next Generaon Gene Sequencing for Solid Tumors Assay Summary IU Health Molecular Pathology Laboratory now offers high throughput sequencing for hot spot mutations found in clinically relevant cancer genes. In addition to a general panel of 48 genes, selected panels have been developed for a more tailored application in specific cancers. Comparing to single gene assay, these panels offer a more comprehensive and economic way to assess prognosis and/or treatment options for cancer patients at the initial diagnosis or at the relapse. Orderable Name: Use IU Health Molecular Pathology requisition; Call 317.491.6417 for requisition. Panels include: Lung cancer panel AKT1, ALK, BRAF, EGFR, KRAS, MET, NRAS, PIK3CA, PTEN Colon cancer panel APC, AKT1, BRAF, KRAS, NRAS, PIK3CA, PTEN, SMAD4 Gastrointestinal stromal tumor (GIST) panel BRAF, KIT, PDGFRA Melanoma panel BRAF, CTNNB1, GNA11, GNAQ, KIT, NRAS Ovarian cancer panel BRAF, KRAS, PIK3CA, PTEN Thyroid cancer panel KIT, BRAF, RET Breast cancer panel AKT1, ERBB2, PIK3CA Oncology sequencing panel ABL1, AKT1, ALK, APC, ATM, BRAF, CDH1, CDKN2A, CSF1R, CTNNB1, EGFR, ERBB2, ERBB4, FBXW7, FGFR1, FGFR2, FGFR3, FLT3, GNA11, GNAQ, GNAS, HNF1A, HRAS, IDH1, JAK2, JAK3, KDR, KIT, KRAS, MET, MLH1, MPL, NOTCH1, NPM1, NRAS, PDGFRA, PIK3CA, PTEN, PTPN11, RB1, RET, SMAD4, SMARCB1, SMO, SRC, STK11, TP53, VHL Clinical Utility: This test is useful for the assessment of prognosis and/or treatment options for individuals with cancers at initial diagnosis or at replase1. Clinical Information: The Oncology Sequencing Solid Tumor Panel is a highly multiplexed targeted resequencing assay for detecting somatic mutations across hundreds of mutational hotspots in cancer genomes. -
"The Genecards Suite: from Gene Data Mining to Disease Genome Sequence Analyses". In: Current Protocols in Bioinformat
The GeneCards Suite: From Gene Data UNIT 1.30 Mining to Disease Genome Sequence Analyses Gil Stelzer,1,5 Naomi Rosen,1,5 Inbar Plaschkes,1,2 Shahar Zimmerman,1 Michal Twik,1 Simon Fishilevich,1 Tsippi Iny Stein,1 Ron Nudel,1 Iris Lieder,2 Yaron Mazor,2 Sergey Kaplan,2 Dvir Dahary,2,4 David Warshawsky,3 Yaron Guan-Golan,3 Asher Kohn,3 Noa Rappaport,1 Marilyn Safran,1 and Doron Lancet1,6 1Department of Molecular Genetics, Weizmann Institute of Science, Rehovot, Israel 2LifeMap Sciences Ltd., Tel Aviv, Israel 3LifeMap Sciences Inc., Marshfield, Massachusetts 4Toldot Genetics Ltd., Hod Hasharon, Israel 5These authors contributed equally to the paper 6Corresponding author GeneCards, the human gene compendium, enables researchers to effectively navigate and inter-relate the wide universe of human genes, diseases, variants, proteins, cells, and biological pathways. Our recently launched Version 4 has a revamped infrastructure facilitating faster data updates, better-targeted data queries, and friendlier user experience. It also provides a stronger foundation for the GeneCards suite of companion databases and analysis tools. Improved data unification includes gene-disease links via MalaCards and merged biological pathways via PathCards, as well as drug information and proteome expression. VarElect, another suite member, is a phenotype prioritizer for next-generation sequencing, leveraging the GeneCards and MalaCards knowledgebase. It au- tomatically infers direct and indirect scored associations between hundreds or even thousands of variant-containing genes and disease phenotype terms. Var- Elect’s capabilities, either independently or within TGex, our comprehensive variant analysis pipeline, help prepare for the challenge of clinical projects that involve thousands of exome/genome NGS analyses.