PACAP System Evolution and Its Role in Melanophore Function in Teleost
Total Page:16
File Type:pdf, Size:1020Kb
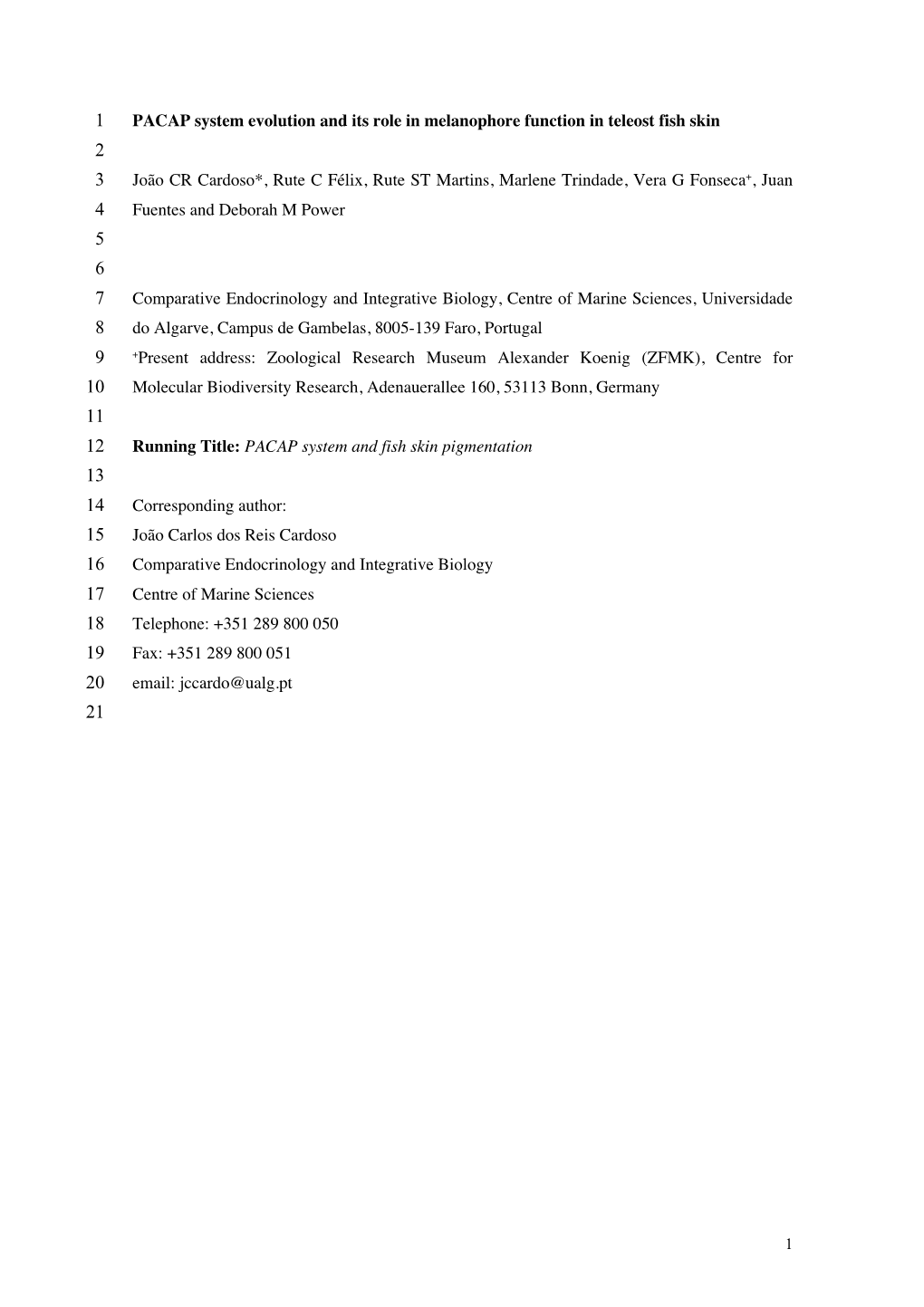
Load more
Recommended publications
-
Adaptive Evolution of Low Salinity Tolerance and Hypoosmotic Regulation in a Euryhaline Teleost, Takifugu Obscurus
Adaptive evolution of low salinity tolerance and hypoosmotic regulation in a euryhaline teleost, Takifugu obscurus Hanyuan Zhang Key Laboratory of Aquatic Genomics, Ministry of Agriculture, CAFS Key Laboratory of Aquatic Genomics and Beijing Key Laboratory of Fishery Biotechnology, Chinese Academy of Fishery Sciences, Fengtai, Beijing, China Jilun Hou Beidaihe Central Experiment Station, Chinese Academy of Fishery Sciences, Qinhuangdao, Hebei, China Haijin Liu Breeding Laboratory, Dalian Tianzheng Industry Co. Ltd., Dalian, Liaoning, China Haoyong Zhu Wuxi Fisheries College, Nanjing Agricultural University, Wuxi, China Gangchun Xu Freshwater Fisheries Research Centre of Chinese Academy of Fishery Sciences, Wuxi, China Jian Xu ( [email protected] ) Chinese Academy of Fishery Sciences https://orcid.org/0000-0003-0274-4268 Research article Keywords: Takifugu, low salt-tolerance, hypoosmotic regulation, population genomics, genome re- sequencing Posted Date: October 30th, 2019 DOI: https://doi.org/10.21203/rs.2.16620/v1 License: This work is licensed under a Creative Commons Attribution 4.0 International License. Read Full License Version of Record: A version of this preprint was published at Marine Biology on June 3rd, 2020. See the published version at https://doi.org/10.1007/s00227-020-03705-x. Page 1/20 Abstract Background The mechanism of osmoregulation is crucial for maintaining growth, development, and life activities in teleosts. Takifugu obscurus, the only euryhaline species in the genus Takifugu, is a proper model organism for studying the mechanism of low salt-tolerance and hypoosmotic regulation. Results In this study, whole genome sequencing data were obtained from 90 puffersh representing ve species within this genus, T. rubripes, T. obscurus, T. -
2. Puffer Fish
Puffer Fish and its Consumption: To Eat or Not to Eat? Inês Panão 1, Conrado Carrascosa 2, José Raduán Jaber 3, António Raposo* 1 1Centro de Investigação Interdisciplinar Egas Moniz, CiiEM, Instituto Superior de Ciências da Saúde Egas Moniz, ISCSEM, Quinta da Granja, Monte de Caparica, 2829-511 Caparica, Portugal. 2Department of Animal Pathology and Production, Bromatology and Food Technology, Faculty of Veterinary, Universidad de Las Palmas de Gran Canaria, Trasmontaña s/n, 35413 Arucas, Spain. 3Department of Morphology, Faculty of Veterinary, Universidad de Las Palmas de Gran Canaria, Trasmontaña s/n, 35413 Arucas, Spain. Corresponding author: António Raposo, E-mail address: [email protected], Phone: (+351)918376093 Abstract This systematic review was done to examine the substantial increase in the number of intoxication cases in puffer fish associated with tetrodotoxin. In the past 5 years, 430 cases of intoxication and 52 deaths associated with puffer fish have been reported worldwide. It has also been verified that puffer fish have migrated to different regions, which has led to a negative Downloaded by [António Raposo] at 05:58 25 July 2015 environmental impact. Based on the information obtained herein, consumption of puffer fish should be legally limited, although it still remains very popular in several regions with negative social and economic impacts. Keywords:Accepted puffer fish; tetrodotoxin; food safety; food-borneManuscript diseases; toxicity. 1 1. Introduction Nowadays, the fish industry is an international business whose global production is estimated at US$ 232 billion in 2012 (1) . Fisheries provide economic and nutritional benefits to people worldwide and are a major source of dietary animal protein (2) . -
Odete Marinho Gonçalves the Origin of Stomach
ODETE MARINHO GONÇALVES THE ORIGIN OF STOMACH VERTEBRATE AND THE PARADOX OF LOSS Tese de Candidatura ao grau de Doutor em Ciências Biomédicas submetida ao Instituto de Ciências Biomédicas Abel Salazar da Universidade do Porto. Orientador – Doutor Jonathan M. Wilson Categoria – Professor auxiliar/Investigador Afiliação – Universidade de Wilfrid Laurier, Waterloo, Canadá/ CIIMAR- Centro Interdisciplinar de Investigação marinha e ambiental. Coorientador – Doutor L. Filipe C. Castro Categoria – Investigador auxiliar Afiliação – CIIMAR- Centro Interdisciplinar de Investigação do mar e ambiente. Coorientador – Professor João Coimbra Categoria – Professor emérito/ Investigador Afiliação – ICBAS- Instituto de Ciências Biomédicas Abel Salazar/ CIIMAR- Centro Interdisciplinar de Investigação marinha e ambiental. This work was conducted in laboratory of Molecular Physiology (MP) at CIIMAR- Centro Interdisciplinar de Investigação Marinha e Ambiental. The animals were maintained at BOGA- Biotério de Organismos Aquáticos. The Chapter 5 of this thesis was partially developed at Station Biologique de Roscoff (France) in laboratory of Dr. Sylvie Mazan (2013). This Chapter was done in collaboration with Dr. Renata Freitas from IBMC. Some work was also developed in Laboratory of Professor Eduardo Rocha at ICBAS- Instituto de Ciências Biomédicas Abel Salazar. This work was funded by FCT scholarship with reference: SFRH/ BD/ 79821/ 2011 “The theories exposed in this work are the exclusive responsibility of the author” AKNOWLEDGMENTS I am grateful to my supervisor Dr. Jonathan Wilson and my co-supervisor Dr. Filipe Castro. This fascinating work begun almost one decade ago (2007) with my master that allow we work together until now. Thanks to Professor João Coimbra to be so kind and to show interested in my work. -
Adaptive Evolution of Low Salinity Tolerance and Hypoosmotic Regulation in a Euryhaline Teleost, Takifugu Obscurus
Adaptive evolution of low salinity tolerance and hypoosmotic regulation in a euryhaline teleost, Takifugu obscurus Hanyuan Zhang Chinese Academy of Fishery Sciences Jilun Hou Chinese Academy of Fishery Sciences Beidaihe Center Experiment Station Haijin Liu Dalian Tianzheng Industry Co. Ltd. Haoyong Zhu Wuxi Fisheries College, Nanjing Agricultural University Gangchun Xu Chinese Academy of Fishery Sciences Freshwater Fisheries Research Center Jian Xu ( [email protected] ) Chinese Academy of Fishery Sciences https://orcid.org/0000-0003-0274-4268 Research article Keywords: Takifugu, low salt-tolerance, hypoosmotic regulation, population genomics, genome re- sequencing Posted Date: August 14th, 2019 DOI: https://doi.org/10.21203/rs.2.12870/v1 License: This work is licensed under a Creative Commons Attribution 4.0 International License. Read Full License Version of Record: A version of this preprint was published at Marine Biology on June 3rd, 2020. See the published version at https://doi.org/10.1007/s00227-020-03705-x. Page 1/20 Abstract Background: The mechanism of osmoregulation is crucial for maintaining growth, development, and life activities in teleosts. Takifugu obscurus, the only euryhaline species in the genus Takifugu, is a proper model organism for studying the mechanism of low salt-tolerance and hypoosmotic regulation. Results: In this study, whole genome sequencing data were obtained from 90 puffersh representing ve species within this genus, T. rubripes, T. obscurus, T. avidus, T. niphobles, and T. bimaculatus. Using a phylogeny, PCA, and population structure analyses, we observed similar amounts of population genetic differentiation among species. The ve species are closely related to each other and have differentiated within a relatively short period, while T. -
Comments on Puffers of the Genus Takifugu from Russian Waters with the First Record of Yellowfin Puffer, Takifugu Xanthopterus (
Bull. Natl. Mus. Nat. Sci., Ser. A, 42(3), pp. 133–141, August 22, 2016 Comments on Puffers of the Genus Takifugu from Russian Waters with the First Record of Yellowfin Puffer, Takifugu xanthopterus (Tetraodontiformes, Tetraodontidae) from Sakhalin Island Yury V. Dyldin1, Keiichi Matsuura2 and Sergey S. Makeev3 1 Tomsk State University, Lenin Avenue 36, Tomsk, 634050, Russia E-mail: [email protected] 2 National Museum of Nature and Science, 4–1–1 Amakubo, Tsukuba, Ibaraki 305–0005, Japan E-mail: [email protected] 3 FGBI Sakhalinrybvod, ul. Emelyanova, 43A, 693000 Yuzhno-Sakhalinsk, Russia E-mail: [email protected] (Received 31 March 2016; accepted 22 June 2016) Abstract In August 2015 a single specimen of Takifugu xanthopterus was collected at the mouth of the Lyutoga River in Aniva Bay, southern Sakhalin Island in the southern Sea of Okhotsk. This is the first discovery of this species from Sakhalin Island, and represents the northernmost record for the species. Also we report eight species of Takifugu from Russia based on newly collected specimens, our survey of literature and the fish collection of the Zoological Institute, Russian Academy of Sciences. Key words : Puffers, Takifugu, taxonomy, Sakhalin, new record Introduction phyreus (Temminck and Schlegel, 1850) and T. rubripes (Temminck and Schlegel, 1850) have Sakhalin Island is the largest island of the Rus- been collected in this area (see Schmidt, 1904; sian Federation and surrounded by the Seas of Lindberg et al., 1997; Sokolovsky et al., 2011). Japan and Okhotsk (Fig. 1). The west coast of During the course of study on the fish fauna of Sakhalin is washed by the warm Tsushima Cur- Sakhalin Island by the first author, specimens of rent and the east coast by the cold East Sakhalin the genus Takifugu have become available for Current. -
The Digestive Tract As an Essential Organ for Water Acquisition in Marine Teleosts: Lessons from Euryhaline Eels Yoshio Takei
Takei Zoological Letters (2021) 7:10 https://doi.org/10.1186/s40851-021-00175-x REVIEW Open Access The digestive tract as an essential organ for water acquisition in marine teleosts: lessons from euryhaline eels Yoshio Takei Abstract Adaptation to a hypertonic marine environment is one of the major topics in animal physiology research. Marine teleosts lose water osmotically from the gills and compensate for this loss by drinking surrounding seawater and absorbing water from the intestine. This situation is in contrast to that in mammals, which experience a net osmotic loss of water after drinking seawater. Water absorption in fishes is made possible by (1) removal of monovalent ions (desalinization) by the − esophagus, (2) removal of divalent ions as carbonate (Mg/CaCO3) precipitates promoted by HCO3 secretion, and (3) facilitation of NaCl and water absorption from diluted seawater by the intestine using a suite of unique transporters. As a result, 70–85% of ingested seawater is absorbed during its passage through the digestive tract. Thus, the digestive tract is an essential organ for marine teleost survival in the hypertonic seawater environment. The eel is a species that has been frequently used for osmoregulation research in laboratories worldwide. The eel possesses many advantages as an experimental animal for osmoregulation studies, one of which is its outstanding euryhalinity, which enables researchers to examine changes in the structure and function of the digestive tract after direct transfer from freshwater to seawater. In recent years, the molecular mechanisms of ion and water transport across epithelial cells (the transcellular route) and through tight junctions (the paracellular route) have been elucidated for the esophagus and intestine. -
Integration of the Genetic Map and Genome Assembly of Fugu Facilitates Insights Into Distinct Features of Genome Evolution in Teleosts and Mammals
GBE Integration of the Genetic Map and Genome Assembly of Fugu Facilitates Insights into Distinct Features of Genome Evolution in Teleosts and Mammals Wataru Kai1, Kiyoshi Kikuchi*,1, Sumanty Tohari2, Ah Keng Chew2, Alice Tay2, Atushi Fujiwara3, Sho Hosoya1, Hiroaki Suetake1, Kiyoshi Naruse4, Sydney Brenner2, Yuzuru Suzuki1, and Byrappa Venkatesh2 1Fisheries Laboratory, Graduate School of Agricultural and Life Sciences, University of Tokyo, Hamamatsu, Shizuoka, Japan 2Institute of Molecular and Cell Biology, A*STAR, Biopolis, Singapore, Singapore 3National Research Institute of Fisheries Science, Fisheries Research Agency, Yokohama, Kanagawa, Japan 4Laboratory of Bioresources, National Institute for Basic Biology, Okazaki, Aichi, Japan *Corresponding author: E-mail: [email protected]. Accepted: 21 April 2011 Abstract The compact genome of fugu (Takifugu rubripes) has been used widely as a reference genome for understanding the evolution of vertebrate genomes. However, the fragmented nature of the fugu genome assembly has restricted its use for comparisons of genome architecture in vertebrates. To extend the contiguity of the assembly to the chromosomal level, we have generated a comprehensive genetic map of fugu and anchored the scaffolds of the assembly to the 22 chromosomes of fugu. The map consists of 1,220 microsatellite markers that provide anchor points to 697 scaffolds covering 86% of the genome assembly (http://www.fugu-sg.org/). The integrated genome map revealed a higher recombination rate in fugu compared with other vertebrates and a wide variation in the recombination rate between sexes and across chromosomes of fugu. We used the extended assembly to explore recent rearrangement events in the lineages of fugu, Tetraodon, and medaka and compared them with rearrangements in three mammalian (human, mouse, and opossum) lineages. -
University of Cape Town
Town Cape Dedicated dads: A study on the nesting ofbehaviour of Spondyliosoma emarginatum (Telostei: Sparidae) Nina Faure Beaulieu FRBNIN001 Dissertation presented for the degree of Master of Science in the Department of Biological UniversitySciences, University of Cape Town October 2020 Supervised by Associate Professor Colin G. Attwood The copyright of this thesis vests in the author. No quotation from it or information derived from it is to be published without full acknowledgement of the source. The thesis is to be used for private study or non- commercial research purposes only. Published by the University of Cape Town (UCT) in terms of the non-exclusive license granted to UCT by the author. University of Cape Town Declaration Name: Nina Faure Beaulieu Student Number: FRBNIN001 Course: MSc Biological Sciences (dissertation only) 1. I know that plagiarism is wrong. Plagiarism is to use another’s work and pretend that it is one’s own. 2. I have used the Harvard referencing style convention for citation and referencing. Each contribution to, and quotation in, this dissertation from the work(s) of other people has been attributed and has been cited and referenced. 3. This dissertation is my own work. 4. I have not allowed, and will not allow, anyone to copy my work with the intention of passing it off as his or her own work. Signature: Date: 09/10/2020 Abstract Fish display the most diverse parental care behaviours within the animal kingdom. These behaviours are important from evolutionary and conservation perspectives as parental care is critical for the development and survival of the young. -
Chromosome Genome Assembly and Annotation of the Yellowbelly
www.nature.com/scientificdata OPEN Chromosome genome assembly Data Descriptor and annotation of the yellowbelly puferfsh with PacBio and Hi-C sequencing data Yitao Zhou1,7, Shijun Xiao2,7, Gang Lin1,3, Duo Chen1, Wan Cen1, Ting Xue1, Zhiyu Liu4, Jianxing Zhong4, Yanting Chen5, Yijun Xiao1, Jianhua Chen1, Yunhai Guo6, Youqiang Chen1, Yanding Zhang1, Xuefeng Hu1* & Zhen Huang 1* Puferfsh are ideal models for vertebrate chromosome evolution studies. The yellowbelly puferfsh, Takifugu favidus, is an important marine fsh species in the aquaculture industry and ecology of East Asia. The chromosome assembly of the species could facilitate the study of chromosome evolution and functional gene mapping. To this end, 44, 27 and 50 Gb reads were generated for genome assembly using Illumina, PacBio and Hi-C sequencing technologies, respectively. More than 13 Gb full-length transcripts were sequenced on the PacBio platform. A 366 Mb genome was obtained with the contig of 4.4 Mb and scafold N50 length of 15.7 Mb. 266 contigs were reliably assembled into 22 chromosomes, representing 95.9% of the total genome. A total of 29,416 protein-coding genes were predicted and 28,071 genes were functionally annotated. More than 97.7% of the BUSCO genes were successfully detected in the genome. The genome resource in this work will be used for the conservation and population genetics of the yellowbelly puferfsh, as well as in vertebrate chromosome evolution studies. Background & Summary Te yellowbelly puferfsh (FishBase ID: 24266), Takifugu favidus, is an economically and ecologically impor- tant fsh species in coastal regions of East Asia, including the East China Sea, Yellow Sea and Bohai Bay1,2. -
Multiple Invasions Into Freshwater by Pufferfishes (Teleostei: Tetraodontidae): a Mitogenomic Perspective
Multiple Invasions into Freshwater by Pufferfishes (Teleostei: Tetraodontidae): A Mitogenomic Perspective Yusuke Yamanoue1*¤, Masaki Miya2, Hiroyuki Doi3, Kohji Mabuchi1, Harumi Sakai4, Mutsumi Nishida1 1 Atmosphere and Ocean Research Institute, University of Tokyo, Kashiwa, Chiba, Japan, 2 Natural History Museum and Institute, Chiba, Chiba, Japan, 3 Shimonoseki Marine Science Museum ‘Kaikyokan,’ Shimonoseki, Yamaguchi, Japan, 4 Department of Applied Aquabiology, National Fisheries University, Shimonoseki, Yamaguchi, Japan Abstract Pufferfishes of the Family Tetraodontidae are the most speciose group in the Order Tetraodontiformes and mainly inhabit coastal waters along continents. Although no members of other tetraodontiform families have fully discarded their marine lives, approximately 30 tetraodontid species spend their entire lives in freshwaters in disjunct tropical regions of South America, Central Africa, and Southeast Asia. To investigate the interrelationships of tetraodontid pufferfishes and thereby elucidate the evolutionary origins of their freshwater habitats, we performed phylogenetic analysis based on whole mitochondrial genome sequences from 50 tetraodontid species and closely related species (including 31 newly determined sequences). The resulting phylogenies reveal that the family is composed of four major lineages and that freshwater species from the different continents are independently nested in two of the four lineages. A monophyletic origin of the use of freshwater habitats was statistically rejected, and ancestral habitat reconstruction on the resulting tree demonstrates that tetraodontids independently entered freshwater habitats in different continents at least three times. Relaxed molecular- clock Bayesian divergence time estimation suggests that the timing of these invasions differs between continents, occurring at 0–10 million years ago (MA) in South America, 17–38 MA in Central Africa, and 48–78 MA in Southeast Asia. -
The Status of Yellow Pufferfish, Xenopterus Naritus (Richardson
The status of yellow pufferfish, Xenopterus naritus (Richardson, 1848) from the Southwest coast of Sarawak, Northwestern Borneo, Malaysia 1 1 2 Ahmad Syafiq Ahmad Nasir, Samsur Mohamad, Mohammed Mohidin 1 Department of Aquatic Science, Faculty of Resource Science and Technology, Universiti Malaysia Sarawak, Kota Samarahan, Sarawak, Malaysia; 2 Fisheries Research Institute Bintawa, Bintawa, Kuching, Sarawak, Malaysia. Corresponding author: A. S. A. Nasir, [email protected] Abstract. The yellow pufferfish, Xenopterus naritus is a commercially important target species for the artisanal fishermen in Sarawak. There is concern over its conservation and population structure. Therefore we provided a baseline data on size distribution, sex ratio, length-weight relationship and condition factor of 65 specimens of this species, landed from May 2015 to July 2014 from eight distinct locations (Kabong, Sematan, Buntal, Paloh, Sadong Jaya, Pusa, Sebangan and Spaoh) representing the Southwest coast of Sarawak waters. Specimens were obtained by hiring local fishermen to catch them and purchasing from fish markets. Generally, males were significantly smaller and lighter than females. Total length, standard length and body weight of combined sexes of X. naritus was found in the size range of 10.6–29.5 cm, 8.8–26.7 cm and 20.16–646.76 g respectively. Male to female sex ratio was 1M:2.8F. Length–weight relationships of X. naritus exhibited positive growth allometry in males but negative growth allometry in females, whereas positive growth allometry in combined sexes. Condition factor in overall specimens was 2.279±0.434 which reflects the healthiness of X. naritus and favorable environmental conditions in Sarawak waters. -
Cage Culture
ISSN 0429-9345 FAO FISHERIES TECHNICAL PAPER 498 Cage aquaculture Regional reviews and global overview $PWFSQIPUP -BSHFTBMNPODBHFTJOUIF3FMPODBWJ'KPSEJOTPVUIFSO$IJMF%4PUP'"0 FAO FISHERIES Cage aquaculture TECHNICAL Regional reviews and global overview PAPER 498 Edited by Matthias Halwart Fishery Resources Officer (Aquaculture) Aquaculture Management and Conservation Service FAO Fisheries and Aquaculture Department Rome, Italy Doris Soto Senior Fishery Resources Officer (Aquatic Resource Management) Aquaculture Management and Conservation Service FAO Fisheries and Aquaculture Department Rome, Italy and J. Richard Arthur FAO Consultant Barriere British Columbia, Canada FOOD AND AGRICULTURE ORGANIZATION OF THE UNITED NATIONS Rome, 2007 The designations employed and the presentation of material in this information product do not imply the expression of any opinion whatsoever on the part of the Food and Agriculture Organization of the United Nations (FAO) concerning the legal or development status of any country, territory, city or area or of its authorities, or concerning the delimitation of its frontiers or boundaries. The mention of specific companies or products of manufacturers, whether or not these have been patented, does not imply that these have been endorsed or recommended by FAO in preference to others of a similar nature that are not mentioned. The views expressed in this information product are those of the author(s) and do not necessarily reflect the views of FAO. ISBN 978-92-5-105801-5 All rights reserved. Reproduction and dissemination of material in this information product for educational or other non-commercial purposes are authorized without any prior written permission from the copyright holders provided the source is fully acknowledged. Reproduction of material in this information product for resale or other commercial purposes is prohibited without written permission of the copyright holders.