Dual -1 Hahn Polynomials: “Classical”
Total Page:16
File Type:pdf, Size:1020Kb
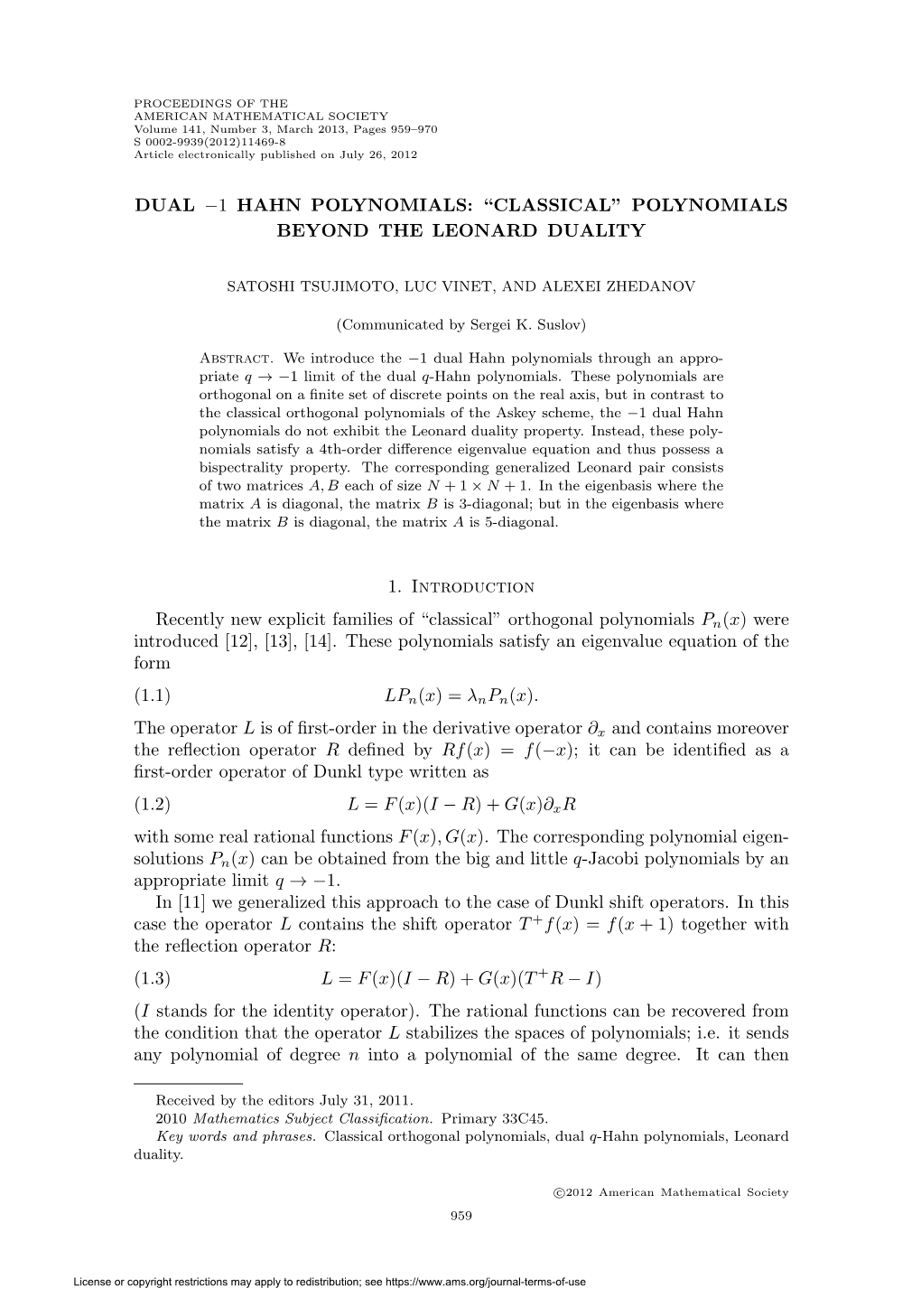
Load more
Recommended publications
-
An Algebraic Interpretation of the Multivariate Q-Krawtchouk Polynomials
An algebraic interpretation of the multivariate q-Krawtchouk polynomials The MIT Faculty has made this article openly available. Please share how this access benefits you. Your story matters. Citation Genest, Vincent X., Sarah Post, and Luc Vinet. “An Algebraic Interpretation of the Multivariate Q-Krawtchouk Polynomials.” The Ramanujan Journal (2016): n. pag. As Published http://dx.doi.org/10.1007/s11139-016-9776-2 Publisher Springer US Version Author's final manuscript Citable link http://hdl.handle.net/1721.1/104790 Terms of Use Article is made available in accordance with the publisher's policy and may be subject to US copyright law. Please refer to the publisher's site for terms of use. Ramanujan J DOI 10.1007/s11139-016-9776-2 An algebraic interpretation of the multivariate q-Krawtchouk polynomials Vincent X. Genest1 · Sarah Post2 · Luc Vinet3 Received: 31 August 2015 / Accepted: 18 January 2016 © Springer Science+Business Media New York 2016 Abstract The multivariate quantum q-Krawtchouk polynomials are shown to arise as matrix elements of “q-rotations” acting on the state vectors of many q-oscillators. The focus is put on the two-variable case. The algebraic interpretation is used to derive the main properties of the polynomials: orthogonality, duality, structure relations, difference equations, and recurrence relations. The extension to an arbitrary number of variables is presented. Keywords Multivariate q-Krawtchouk polynomials · q-Oscillator algebra · q-Rotations Mathematics Subject Classification 33D45 · 16T05 1 Introduction The purpose of this paper is to provide an algebraic model for the multi-variable q- Krawtchouk polynomials and to show how this model provides a cogent framework VXG is supported by a postdoctoral fellowship from the Natural Sciences and Engineering Research Council of Canada (NSERC). -
Journal of Computational and Applied Mathematics Extensions of Discrete
View metadata, citation and similar papers at core.ac.uk brought to you by CORE provided by Elsevier - Publisher Connector Journal of Computational and Applied Mathematics 225 (2009) 440–451 Contents lists available at ScienceDirect Journal of Computational and Applied Mathematics journal homepage: www.elsevier.com/locate/cam Extensions of discrete classical orthogonal polynomials beyond the orthogonality R.S. Costas-Santos a,∗, J.F. Sánchez-Lara b a Department of Mathematics, University of California, South Hall, Room 6607 Santa Barbara, CA 93106, USA b Universidad Politécnica de Madrid, Escuela Técnica Superior de Arquitectura, Departamento de Matemática Aplicada, Avda Juan de Herrera, 4. 28040 Madrid, Spain article info a b s t r a c t α,β Article history: It is well-known that the family of Hahn polynomials fhn .xI N/gn≥0 is orthogonal with Received 5 November 2007 respect to a certain weight function up to degree N. In this paper we prove, by using the three-term recurrence relation which this family satisfies, that the Hahn polynomials can MSC: be characterized by a ∆-Sobolev orthogonality for every n and present a factorization for 33C45 Hahn polynomials for a degree higher than N. 42C05 We also present analogous results for dual Hahn, Krawtchouk, and Racah polynomials 34B24 and give the limit relations among them for all n 2 N0. Furthermore, in order to get Keywords: these results for the Krawtchouk polynomials we will obtain a more general property of Classical orthogonal polynomials orthogonality for Meixner polynomials. Inner product involving difference ' 2008 Elsevier B.V. All rights reserved. -
The Askey-Scheme of Hypergeometric Orthogonal Polynomials and Its Q-Analogue
The Askey-scheme of hypergeometric orthogonal polynomials and its q-analogue Roelof Koekoek Ren´eF. Swarttouw Abstract We list the so-called Askey-scheme of hypergeometric orthogonal polynomials and we give a q- analogue of this scheme containing basic hypergeometric orthogonal polynomials. In chapter 1 we give the definition, the orthogonality relation, the three term recurrence rela- tion, the second order differential or difference equation, the forward and backward shift operator, the Rodrigues-type formula and generating functions of all classes of orthogonal polynomials in this scheme. In chapter 2 we give the limit relations between different classes of orthogonal polynomials listed in the Askey-scheme. In chapter 3 we list the q-analogues of the polynomials in the Askey-scheme. We give their definition, orthogonality relation, three term recurrence relation, second order difference equation, forward and backward shift operator, Rodrigues-type formula and generating functions. In chapter 4 we give the limit relations between those basic hypergeometric orthogonal poly- nomials. Finally, in chapter 5 we point out how the ‘classical’ hypergeometric orthogonal polynomials of the Askey-scheme can be obtained from their q-analogues. Acknowledgement We would like to thank Professor Tom H. Koornwinder who suggested us to write a report like this. He also helped us solving many problems we encountered during the research and provided us with several references. Contents Preface 5 Definitions and miscellaneous formulas 7 0.1 Introduction . 7 0.2 The q-shifted factorials . 8 0.3 The q-gamma function and the q-binomial coefficient . 10 0.4 Hypergeometric and basic hypergeometric functions . -
Doubling (Dual) Hahn Polynomials: Classification and Applications
Symmetry, Integrability and Geometry: Methods and Applications SIGMA 12 (2016), 003, 27 pages Doubling (Dual) Hahn Polynomials: Classification and Applications Roy OSTE and Joris VAN DER JEUGT Department of Applied Mathematics, Computer Science and Statistics, Ghent University, Krijgslaan 281-S9, B-9000 Gent, Belgium E-mail: [email protected], [email protected] Received July 13, 2015, in final form January 04, 2016; Published online January 07, 2016 http://dx.doi.org/10.3842/SIGMA.2016.003 Abstract. We classify all pairs of recurrence relations in which two Hahn or dual Hahn polynomials with different parameters appear. Such couples are referred to as (dual) Hahn doubles. The idea and interest comes from an example appearing in a finite oscillator model [Jafarov E.I., Stoilova N.I., Van der Jeugt J., J. Phys. A: Math. Theor. 44 (2011), 265203, 15 pages, arXiv:1101.5310]. Our classification shows there exist three dual Hahn doubles and four Hahn doubles. The same technique is then applied to Racah polynomials, yielding also four doubles. Each dual Hahn (Hahn, Racah) double gives rise to an explicit new set of symmetric orthogonal polynomials related to the Christoffel and Geronimus trans- formations. For each case, we also have an interesting class of two-diagonal matrices with closed form expressions for the eigenvalues. This extends the class of Sylvester{Kac matrices by remarkable new test matrices. We examine also the algebraic relations underlying the dual Hahn doubles, and discuss their usefulness for the construction of new finite oscillator models. Key words: Hahn polynomial; Racah polynomial; Christoffel pair; symmetric orthogonal polynomials; tridiagonal matrix; matrix eigenvalues; finite oscillator model 2010 Mathematics Subject Classification: 33C45; 33C80; 81R05; 81Q65 1 Introduction The tridiagonal (N + 1) × (N + 1) matrix of the following form 0 0 1 1 BN 0 2 C B C B N − 1 0 3 C C = B C (1.1) N+1 B . -
Spectral Transformations and Generalized Pollaczek Polynomials*
METHODS AND APPLICATIONS OF ANALYSIS. © 1999 International Press Vol. 6, No. 3, pp. 261-280, September 1999 001 SPECTRAL TRANSFORMATIONS AND GENERALIZED POLLACZEK POLYNOMIALS* OKSANA YERMOLAYEVAt AND ALEXEI ZHEDANOV* To Richard Askey on his 65th birthday Abstract. The Christoffel and the Geronimus transformations of the classical orthogonal poly- nomials of a discrete variable are exploited to construct new families of the generalized Pollaczek polynomials. The recurrence coefficients wn, bn of these polynomials are rational functions of the argument n. The (positive) weight function is known explicitly. These polynomials are shown to belong to a subclass of the semi-classical orthogonal polynomials of a discrete variable. We illustrate the method by constructing a family of the modified Charlier polynomials which are orthogonal with respect to a perturbed Poisson distribution. The generating function of these polynomials provides a nontrivial extension of the class of the Meixner-Sheffer generating functions. 1. Introduction. The formal orthogonal polynomials Pn(x) are defined through the three-term recurrence relation ([8]) (1.1) iVfiOc) + unPn-i(x) + bnPn(x) = xPn(x) with the initial conditions (1.2) Po(x) = l, Pi(x)=x-bo. n n_1 The polynomials Pn(x) are monic (i.e. Pn{x) = x 4- 0(a; )). It can be shown that for arbitrary complex coefficients bn and (non-zero) un there exists a linear functional C such that k (1.3) £{Pn(x)x } = hn5nk, k<n, where (1.4) hn - u1U2"'Un ^ 0 are normalization constants. The functional £ is defined on the space of polynomials by its moments n (1.5) cn = £{x }, n = 0,l,---. -
Charting the $ Q $-Askey Scheme
Charting the q-Askey scheme Tom H. Koornwinder, [email protected] Dedicated to Jasper Stokman on the occasion of his fiftieth birthday, in admiration and friendship Abstract Following Verde-Star, Linear Algebra Appl. 627 (2021), we label families of orthogonal polynomials in the q-Askey scheme together with their q-hypergeometric representations by k three sequences xk,hk,gk of Laurent polynomials in q , two of degree 1 and one of degree 2, satisfying certain constraints. This gives rise to a precise classification and parametrization of these families together with their limit transitions. This is displayed in a graphical scheme. We also describe the four-manifold structure underlying the scheme. 1 Introduction The Askey scheme [1, p.46], [7, p.184] and the q-Askey scheme [7, p.414] display in a graphical way the families of (q-)hypergeomtric orthogonal polynomials as they occur as limit cases of the four-parameter top level families: Wilson and Racah polynomials for the Askey scheme and Askey–Wilson and q-Racah polynomials for the q-Askey scheme. By each arrow to the next lower level one parameter is lost. The bottom level families no longer depend on parameters. Since their introduction these schemes have been of great assistance to everybody who needs to do work with one or more of the families in the scheme. These schemes are also expected and partially proven to exist in other contexts, parallel to the original schemes or generalizing them. These contexts are: (i) (q-)hypergeometric biorthog- onal rational functions [3]; (ii) the nonsymmetric case [11], [12]; (iii) the q = −1 case starting arXiv:2108.03858v1 [math.CA] 9 Aug 2021 with the Bannai–Ito polynomials [2, pp. -
Leonard Systems and Their Friends Jonathan Spiewak
University of South Florida Scholar Commons Graduate Theses and Dissertations Graduate School March 2016 Leonard Systems and their Friends Jonathan Spiewak Follow this and additional works at: http://scholarcommons.usf.edu/etd Part of the Mathematics Commons Scholar Commons Citation Spiewak, Jonathan, "Leonard Systems and their Friends" (2016). Graduate Theses and Dissertations. http://scholarcommons.usf.edu/etd/6145 This Thesis is brought to you for free and open access by the Graduate School at Scholar Commons. It has been accepted for inclusion in Graduate Theses and Dissertations by an authorized administrator of Scholar Commons. For more information, please contact [email protected]. Leonard Systems and their Friends by Jonathan Spiewak A thesis submitted in partial fulfillment of the requirements for the degree of Master of Arts Department of Mathematics College of Arts and Sciences University of South Florida Major Professor: Brian Curtin, Ph.D. Brendan Nagle, Ph.D. Jean-Fran¸coisBiasse, Ph.D. Date of Approval: March 2, 2016 Keywords: Leonard pair, Split decomposition, equitable sl 2 basis, equitable Uq(sl 2) generators Copyright c 2016, Jonathan Spiewak Acknowledgements I would like to sincerely thank Dr. Brian Curtin for his knowledge, guidance, and patience. I would also like to thank the Department of Mathematics at University of South Florida, my thesis committee, and Dr. Ana Torres for her interest and support. I would like to thank my parents, for believing in me and always encouraging me to aspire high. I would like to thank my husband, Kenny, who kept me focused and motivated throughout this entire process. Table of Contents List of Tables . -
Orthogonal Polynomials and Classical Orthogonal Polynomials
International Journal of Mechanical Engineering and Technology (IJMET) Volume 9, Issue 10, October 2018, pp. 1613–1630, Article ID: IJMET_09_10_164 Available online at http://iaeme.com/Home/issue/IJMET?Volume=9&Issue=10 ISSN Print: 0976-6340 and ISSN Online: 0976-6359 © IAEME Publication Scopus Indexed ORTHOGONAL POLYNOMIALS AND CLASSICAL ORTHOGONAL POLYNOMIALS DUNIA ALAWAI JARWAN Education for Girls College, Al-Anbar University, Ministry of Higher Education and Scientific Research, Iraq ABSTRACT The focus of this project is to clarify the concept of orthogonal polynomials in the case of continuous internal and discrete points on R and the Gram – Schmidt orthogonalization process of conversion to many orthogonal limits and the characteristics of this method. We have highlighted the classical orthogonal polynomials as an example of orthogonal polynomials because of they are great importance in physical practical applications. In this project, we present 3 types (Hermite – Laguerre – Jacobi) of classical orthogonal polynomials by clarifying the different formulas of each type and how to reach some formulas, especially the form of the orthogonality relation of each. Keywords: Polynomials, Classical Orthogonal, Monic Polynomial, Gram – Schmidt Cite this Article Dunia Alawai Jarwan, Orthogonal Polynomials and Classical Orthogonal Polynomials, International Journal of Mechanical Engineering and Technology, 9(10), 2018, pp. 1613–1630. http://iaeme.com/Home/issue/IJMET?Volume=9&Issue=10 1. INTRODUCTION The mathematics is the branch where the lots of concepts are included. An orthogonality is the one of the concept among them. Here we focuse on the orthogonal polynomial sequence. The orthogonal polynomial are divided in two classes i.e. classical orthogonal polynomials, Discrete orthogonal polynomials and Sieved orthogonal polynomials .There are different types of classical orthogonal polynomials such that Jacobi polynomials, Associated Laguerre polynomials and Hermite polynomials. -
Q-Hermite Polynomials and Classical Orthogonal Polynomials ∗
Q-Hermite Polynomials and Classical Orthogonal Polynomials ∗ Christian Berg and Mourad E. H. Ismail February 17, 1995 Abstract We use generating functions to express orthogonality relations in the form of q-beta integrals. The integrand of such a q-beta integral is then used as a weight function for a new set of orthogonal or biorthogonal functions. This method is applied to the continuous q-Hermite polynomials, the Al-Salam-Carlitz polynomials, and the polynomials of Szeg˝o and leads naturally to the Al-Salam-Chihara polynomials then to the Askey-Wilson poly- nomials, the big q-Jacobi polynomials and the biorthogonal rational functions of Al-Salam and Verma, and some recent biorthogonal functions of Al-Salam and Ismail. Running title: Classical Orthogonal Polynomials. 1990 Mathematics Subject Classification: Primary 33D45, Secondary 33A65, 44A60. em Key words and phrases. Askey-Wilson polynomials, q-orthogonal polynomials, orthogo- nality relations, q-beta integrals, q-Hermite polynomials, biorthogonal rational functions. 1. Introduction and Preliminaries. The q-Hermite polynomials seem to be at the bottom of a hierarchy of the classical q-orthogonal polynomials, [6]. They contain no parameters, other than q, and one can get them as special or limiting cases of other orthogonal polynomials. The purpose of this work is to show how one can systematically build the classical q-orthogonal polynomials from the q-Hermite polynomials using a simple procedure of attaching generating functions to measures. Let p (x) be orthogonal polynomials with respect to a positive measure µ with moments { n } of any order and infinite support such that ∗Research partially supported by NSF grant DMS 9203659 1 ∞ (1.1) pn(x)pm(x) dµ(x)= ζnδm,n. -
Moments of Orthogonal Polynomials and Exponential Generating
MOMENTS OF ORTHOGONAL POLYNOMIALS AND EXPONENTIAL GENERATING FUNCTIONS IRA M. GESSEL AND JIANG ZENG Dedicated to the memory of Richard Askey Abstract. Starting from the moment sequences of classical orthogonal polynomials we derive the orthogonality purely algebraically. We consider also the moments of (q = 1) classical orthogonal polynomials, and study those cases in which the expo- nential generating function has a nice form. In the opposite direction, we show that the generalized Dumont-Foata polynomials with six parameters are the moments of rescaled continuous dual Hahn polynomials. 1. Introduction Many of the most important sequences in enumerative combinatorics—the factorials, derangement numbers, Bell numbers, Stirling polynomials, secant numbers, tangent numbers, Eulerian polynomials, Bernoulli numbers, and Catalan numbers—arise as moments of well-known orthogonal polynomials. With the exception of the Bernoulli and Catalan numbers, these orthogonal polynomials are all Sheffer type, see [39, 42]. One characteristic of these sequences is that their ordinary generating functions have simple continued fractions. For some recent work on the moments of classical orthog- onal polynomials we refer the reader to [9, 14, 32, 10, 11, 23]. There is another sequence which appears in a number of enumerative applications, and which also has a simple continued fraction. The Genocchi numbers may be defined by arXiv:2107.00255v2 [math.CA] 9 Jul 2021 ∞ xn ∞ x2n+2 x G = G = x tan . n n! 2n+2 (2n + 2)! 2 n=0 n=0 X X So Gn = 0 when n is odd or n = 0, G2 = 1, G4 = 1, G6 = 3, G8 = 17, G10 = 155, and G12 = 2073. -
Moments of Classical Orthogonal Polynomials
Moments of Classical Orthogonal Polynomials zur Erlangung des akademischen Grades eines Doktors der Naturwissenschaften (Dr.rer.nat) im Fachbereich Mathematik der Universität Kassel By Patrick Njionou Sadjang ????? Ph.D thesis co-supervised by: Prof. Dr. Wolfram Koepf University of Kassel, Germany and Prof. Dr. Mama Foupouagnigni University of Yaounde I, Cameroon October 2013 Tag der mündlichen Prüfung 21. Oktober 2013 Erstgutachter Prof. Dr. Wolfram Koepf Universität Kassel Zweitgutachter Prof. Dr. Mama Foupouagnigni University of Yaounde I Abstract The aim of this work is to find simple formulas for the moments mn for all families of classical orthogonal polynomials listed in the book by Koekoek, Lesky and Swarttouw [30]. The generating functions or exponential generating functions for those moments are given. To my dear parents Acknowledgments Foremost, I would like to express my sincere gratitude to my advisors Prof. Dr. Wolfram Koepf and Prof. Dr. Mama Foupouagnigni for the continuous support of my Ph.D study and research, for their patience, motivation, enthusiasm, and immense knowledge. Their guidance helped me in all the time of research and writing of this thesis. I could not have imagined having better advisors and mentors for my Ph.D study. I am grateful to Prof. Dr. Mama Foupouagnigni for enlightening me the first glance of re- search. My sincere thanks also go to Prof. Dr. Wolfram Koepf for offering me the opportunity to visit the University of Kassel where part of this work has been written. I acknowledge the financial supports of the DAAD via the STIBET fellowship which en- abled me to visit the Institute of Mathematics of the University of Kassel. -
Hypergeometric Orthogonal Polynomials and Their Q-Analogues
Springer Monographs in Mathematics Hypergeometric Orthogonal Polynomials and Their q-Analogues Bearbeitet von Roelof Koekoek, Peter A Lesky, René F Swarttouw, Tom H Koornwinder 1. Auflage 2010. Buch. xix, 578 S. Hardcover ISBN 978 3 642 05013 8 Format (B x L): 15,5 x 23,5 cm Gewicht: 2230 g Weitere Fachgebiete > Mathematik > Mathematische Analysis Zu Leseprobe schnell und portofrei erhältlich bei Die Online-Fachbuchhandlung beck-shop.de ist spezialisiert auf Fachbücher, insbesondere Recht, Steuern und Wirtschaft. Im Sortiment finden Sie alle Medien (Bücher, Zeitschriften, CDs, eBooks, etc.) aller Verlage. Ergänzt wird das Programm durch Services wie Neuerscheinungsdienst oder Zusammenstellungen von Büchern zu Sonderpreisen. Der Shop führt mehr als 8 Millionen Produkte. Contents Foreword .......................................................... v Preface ............................................................ xi 1 Definitions and Miscellaneous Formulas ........................... 1 1.1 Orthogonal Polynomials . 1 1.2 The Gamma and Beta Function . 3 1.3 The Shifted Factorial and Binomial Coefficients . 4 1.4 Hypergeometric Functions . 5 1.5 The Binomial Theorem and Other Summation Formulas . 7 1.6 SomeIntegrals............................................. 8 1.7 TransformationFormulas.................................... 10 1.8 The q-ShiftedFactorial...................................... 11 1.9 The q-Gamma Function and q-Binomial Coefficients . 13 1.10 Basic Hypergeometric Functions . 15 1.11 The q-Binomial Theorem and Other Summation Formulas