Wyoming Water Research Program Annual Technical Report FY 2009
Total Page:16
File Type:pdf, Size:1020Kb
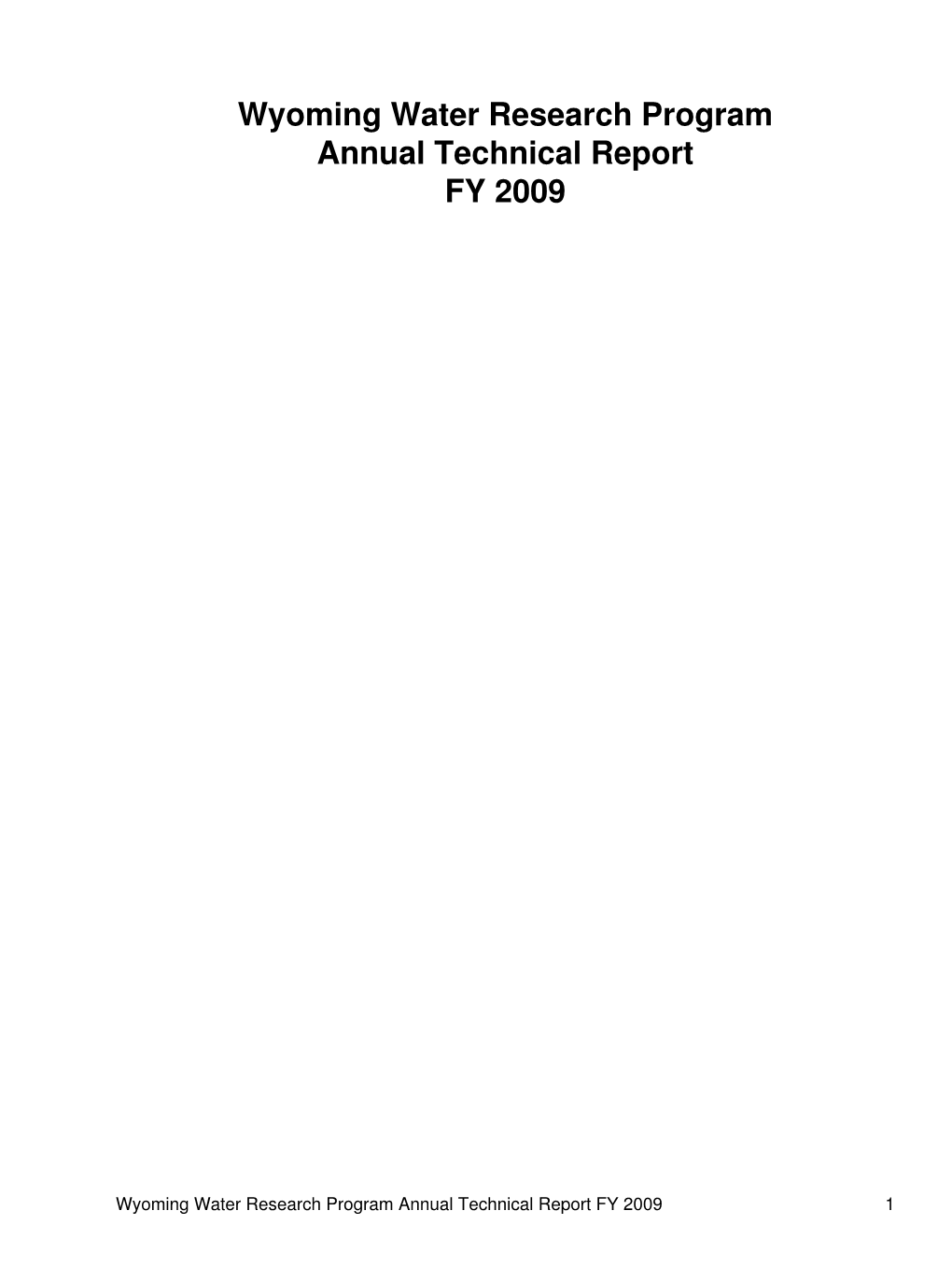
Load more
Recommended publications
-
Generation One 1. John Walden Meyers #80201, B. 22 January
Family of John Walden Meyers and Mary Kruger compiled by John A. Brebner for the Friends of Sandbanks 26th October, 2020 Generation One 1. John Walden Meyers #80201, b. 22 January 1745 in Albany County, New York State,1 occupation Military Captain,2 d. 22 November 1821 in Sidney Township, Hastings County, Ontario,1 buried in Whites Cemetery/St. Thomas Cemetery, Belleville.1 . Pioneer Life on the Bay of Quinte, The Meyers Family, pages 20 - 30 "In an old burial ground on an eminence overlooking the Bay, midway between Belleville and Trenton, where lie the ashes of many of the men who built the first log cabins along the front of Sidney, rest the remains of Captain John Walten (sic) Meyers, the founder of Belleville, and the man who erected the first mills in the County of Hastings. Family tradition has it that the old Captain was born in Prussia. Some years before the American Colonies threw off their allegiance, his father left the land of his birth and came with his family to the colony of New York, where he settled on a farm near Poughkeepsie. The family prospered and were in comfortable circumstances when the war broke out. John Walten, our pioneer, had married Polly Kruger, also a native of Prussia; their children were all born on the homestead in New York, where they remained with their mother throughout the war. John's father and other members of his family cast their lots with the rebels, but John himself remained loyal. He took part in with the Tories in organizing a company for service, but being greatly outnumbered by the Revolutionists, they were. -
Vanalstine #113098. He Married (Unidentified) #113099. Children
Family of Pewter Vanalstine and Alida Vanallen compiled by John A. Brebner for the Friends of Sandbanks 26th October, 2020 Generation One 1. (unidentified) Vanalstine #113098. He married (unidentified) #113099. Children: 2. i. Peter Vanalstine #80096 b. 1747. 3. ii. Cornelius Vanalstine #113100. Generation Two 2. Peter Vanalstine #80096, b. 1747 in Kinderhook, Columbia County, New York, occupation 1775 Blacksmith, occupation Militia Captain, Major, Magistrate, religion Presbyterian (supposedly), d. 02 October 1812 in Adolphustown, Lennox and Addington, Ontario,1 buried in Loyalist Burying Ground, Adolphustown, Lennox and Addington, Ontario. Peter's ancestors emigrated from the Netherlands to New Amsterdam (New York) around 1640. Supported Loyalist cause with General Burgoyne. After that defeat in 1777, and the confiscation of his New York properties, he took refuge in Canada. He returned to British controlled New York City in 1778, where he was awarded the rank of Major with the Associated Loyalists. His family joined him there, and in 1783 they fled, first to Sorel, Quebec via the Hudson River and the Atlantic coast to the St. Lawrence River, and on to Sorel. His wife Alida died at Sorel in 1784. Interestingly, the Vanalstine party appears to have been the only group of Loyalists from New York that chose the sea route from New York, up the coast and up the Saint Lawrence to Sorel. Most of the other Loyalists to Upper Canada chose the inland route; up the Hudson to Albany, the Mohawk and its tributaries to Oswego, and thence across Lake Ontario to Kingston and Prince Edward County. The major advantage of this water passage was that these immigrants could carry far more personal possessions than those relying on the rudimentary trails of the overland route. -
To Founding of WI
A Motheris Tragedy Led. To Founding of WI. PBOGBAMME’. Adelaide Hoodless was a to be portrayed in the histori OF THE woman with a vision who ctal pageant, Portraits From in‘e turned a personal tragedy the Past, to be presented SIDNEY SOUTH into a crusade to raise the B01 and VS auditorium out: ' standards of homemaking March 6' at 8.30 p.m. Mrs. Hoodless was a wo- BRANCH W. I. ’4qu throughout Canada. Little did culture and great she realize that her viSion man of HASTINGS WEST personal charm with a know- would grow to a mighty or- gained during an im-i ganization, the Associated ledge, poverished childhood, of the \ Country Women of‘the World, problems of farm women. ‘ comprising Women's Institut- Born near Brantford in 1857, es and similar organizations youngest of a family of 12, of country women in 108 in 1881 and mov- countries. she married Creek, near Adelaide Hoodless She is one of the characters ed to Stoney, Hamilton. There her four ‘3 children were born, and there SEASON 1967 - 1968 her youngest, a .son born in 1888, died in anfancy as the W e | c o m e I result of a disease transmitt- ‘ ed through contaminated MEETING FIRST WEDNESDAY NIGHT I milk. AT 8:15 O'CLOCK It was her remorse at her ,4 lack of knowledge of child care that stirred Mrs. Hood- OPENING ODE less to a crusade to save A goodly thing it is to meet other mothers and babies In friendships circle bright, 3A,, 3 from the same fate. -
SAMPLE Content Directory
SAMPLE Nearly 1,300 Journals Content Directory Participating! http://heinonline.org Please Note: This is a list of ALL content available in our two main library modules through the HeinOnline interface. Due to the various subscription packages available, access to this content may vary. TABLE OF CONTENTS: Law Journal Library ..................................................................................................................................... pp. 1-25 Legal Classics Library................................................................................................................................. pp. 26-56 LAW JOURNAL LIBRARY VOLUMES YEARS Acta Juridica 1958-2003 1958-2003 Acta Universitatis Lucian Blaga 2001-2005 2001-2005 Adelaide Law Review 1-24 1960-2003 Administrative Law Journal of the American University† 1-10 1987-1996 Administrative Law Review (ABA) 1-57 1949-2005 Advocate: A Weekly Law Journal† 1-2 1888-1890 African Human Rights Law Journal 1-3 2001-2003 African Journal of Legal Studies 1 2004-2005 Air Force Law Review 1-58 1959-2006 Air Law Review † 1-12 1930-1941 Akron Law Review 1-38 1967-2005 Akron Tax Journal 1-21 1983-2006 Alabama Law Journal (Birmingham) † 1-5 1925-1930 Alabama Law Journal (Montgomery) † 1-4 1882-1885 Alabama Law Review *JUST UPDATED* 1-57 1948-2006 Alaska Law Review 1-22 1984-2005 Albany Law Environmental Outlook Journal 1-10 1995-2005 Albany Law Journal † 1-70 1870-1909 Albany Law Journal of Science and Technology 1-15 1991-2005 Albany Law Review 1-69 1931-2006 Alberta Law Quarterly -
694 STATISTICAL YEAR-BOOK the Following Are the Lists of The
694 STATISTICAL YEAR-BOOK The following are the lists of the members of the several Provincial Legislatures :— PROVINCE OF ONTARIO. LEGJSLATIVE ASSEMBLY, 1903. SPEAKER—Hox. WILLIAM A. CHARLTON. CLEKK—CHAS. CLARKE. Constituencies. Representatives. Constituencies, Representatives. Addington Reid, James Middlesex, West. Ross, Hon. Geo. W. Algoma Smyth, W. R. Monck Harconrt, Hon. R. Brant, North Burt, Daniel Muskoka Vacant. Brant, South Preston, Thomas H. Nipissing, West.. Michaud, Joseph Brockville Graham, Geo. P. Ni pissing, East.. James, M. Bruce, Centre.... Clark, Hugh Norfolk, North .. Little, Archibald Bruce, North Bowman, Chas. M. Norfolk, South. Charlton, Hon. W. A. Bruce, South Truax, R. A. NorthumbTnd,E. Wilkmghby, William A. Cardwell Little, E. A. Northumb'l'nd, W Clarke, Samuel Carleton Kidd, G. N. Ontario, North .. Hoyle, W. H. Dufferin Barr, John Ontario, South... Dryden, Hon. J. Dundas Whitney, J. P. f Murphy, Dennis Durham, East.... Preston, Josiah Ottawa. Powell, C. B. Durham, West... Rickard, William Oxford, North... Pattullo, Andrew Elgin, East Brower, C. A. Oxford, South.... Sutherland, D. Elgin, West Macdiarmid, Finlay G. Parry Sound Carr, Milton Essex, North Reaunie, Joseph C. Peel Smith, J. Essex, South Auld. John Allan Perth, North .... Brown, John. Fort William and Perth, South Stock, Valentine Lake of the Woods Cameron, D. C. Peterborough, E. Anderson, William. Frontenac Gallagher, John S. Peterborough, W. Stratton,Hon. J. R. Glengarry McLeod, Wm. D. Port Arthnr and Grenville Joynt, R. L. Rainy River ... Conmee, James Grey, Centre Lucas, J. B. Prescott Evanturel, Hon. F. E. A. Grey, North Boyd, G. M. Prince Edward... Currie, Morley Grey. South Jamieson, D. Renfrew, North.. Vacant. -
Dual-Economy Growth, Trade, and Development
STRUCTURAL CHANGE AND INCOME DIFFERENCES by Trevor Tombe A thesis submitted in conformity with the requirements for the degree of Doctor of Philosophy Graduate Department of Economics University of Toronto Copyright c 2011 by Trevor Tombe Abstract Structural Change and Income Differences Trevor Tombe Doctor of Philosophy Graduate Department of Economics University of Toronto 2011 Economic growth and development is intimately related to the decline of agriculture’s share of output and employment. This process of structural change has important implications for income and pro- ductivity differences between regions within a country or between countries themselves. Agriculture typically has low productivity relative to other sectors and this is particularly true in poor areas. So, as labour switches to nonagricultural activities or as agricultural productivity increases, poor agriculturally- intensive areas will benefit the most. In this thesis, I contribute to a recent and growing line of research and incorporate a separate role for agriculture, both into modeling frameworks and data analysis, to examine income and productivity differences. I first demonstrate that restrictions on trade in agricultural goods, which support inefficient domestic producers, inhibit structural change and lower productivity in poor countries. To do this, I incorporate multiple sectors, non-homothetic preferences, and labour mobility costs into an Eaton-Kortum trade model. With the model, I estimate productivity from trade data (avoiding problematic data for poor countries that typical estimates require) and perform a variety of counterfactual exercises. I find im- port barriers and labour mobility costs account for one-third of the aggregate labour productivity gap between rich and poor countries and for nearly half the gap in agriculture. -
Historic West Hastings Map Guide
HISTORIC WEST HASTINGS MAP GUIDE www.vancouverheritagefoundation.org Introduction This map guide focuses on the western section of Hastings Street, west of Victory Square. Equalled in importance only by Granville Street, Hastings has been a part of every phase of Vancouver’s history. In the city’s early years, Hastings and Main was the principal cross- roads. Today, the nearby convention centre, Waterfront Station and SFU campus ensure the importance of Hastings Street’s western end. The city’s retail centre moved west along Hastings in the 1900s, gradually abandoning East Hastings between Cambie and Dunlevy to low-end shops and hotels. The coup de grâce for this eastern part was the move in 1957 by the BC Electric Company from its head office building at Carrall and Hastings to a new office building at Nelson and Burrard (now The Electra condominiums); with the closure of both the interurban railway system, which had terminated at Carrall, and the north shore ferry service that docked at the foot of Columbia, there was little pedestrian traffic to support local businesses. The prestigious residential district once known as Blueblood Alley west of Granville became commercial beginning in the 1900s; high-end residential began to return in the 2000s in very different types of buildings, reflecting the redevelopment of the Coal Harbour shore- line with highrise condominiums. A chronology of West Hastings: Before 1886: First Nations people had a village at Khwaykhway (Lumbermen’s Arch) in Stanley Park and a handful of ship-jumpers and pioneers settled in small homes along Coal Harbour. John Morton, one of the “Three Greenhorns” who pre-empted District Lot 185 (the West End), built a cabin on the bluff near the foot of Thurlow Street in 1862. -
Mayors Database
MAYORS OF THE CITY OF BELLEVILLE A story of the Mayors of Belleville, Ontario, 1850-2003, with some associated genealogy and 19th & 20th century advertisements. Dr. Donald Brearley Published by the Quinte Branch, Ontario Genealogical Society, 2011 Last update: August 2016 Mayors of the City of Belleville, Ontario, 1850-2003 by Dr. Donald Brearley __________________ CONTENTS INTRODUCTION ............................................................................................. 5 About the author, Dr. Donald Brearley ................................................... 5 The City of Belleville.............................................................................. 5 DAVY, Benjamin Fairfield (1804-1860) .......................................................... 6 PONTON, William Hamilton (1810-1890) ....................................................... 6 O’HARE, John (1825-1865) ............................................................................. 7 McANNANY, Francis (c1805-1877) ................................................................ 8 HOPE, William M.D.(1815-1894) .................................................................... 9 BROWN, James (1823-1897) ......................................................................... 10 HOLDEN, Rufus M.D (1809-1876) ................................................................ 10 FLINT, Billa (1805-1894) ............................................................................... 11 CORBY, Henry (1806-1881) ......................................................................... -
Appointments to the Senate, Necrology and By-Elections
78 STATISTICAL YEAR-BOOK APPOINTMENTS TO THE SENATE. Hon. Robert Mackay, Quebec, 21st January, 1901. " A. T. Wood, Ontario, 21st January, 1901. " Lyman M. Jones, Ontario, 21st January, 1901. " George McHugh, Ontario, 21st January, 1901. " George Landerkin, M.D., Ontario, 16th February, 1901. " Joseph Godbout, Quebec, 4th April, 1901. " Arthur M. Dechene, Quebec, 13th May, 1901. " James E. Robertson, M.D., Prince Edward Island, 8th February, 1902. " Charles E. Church, Nova Scotia, 8th February, 1902. " Frederick P. Thompson, New Brunswick, 8th February, 1902. " Frederic L. Beique, Quebec, 11th February, 1902. " William Gibson, Ontario, 11th February, 1902. " James McMullen, Ontario, 8th February, 1902. NECROLOGY. Hon. Frank Smith, Senator, Ontario, 17th January, 1901. " G. C. McKindsey, Senator, Ontario, 12th February, 1901. " W. J. Almon, Nova Scotia, 18th February, 1901. " Joseph A. Paquet, Senator, Quebec, 29th March, 1901. " John J. Ross, Senator, Quebec, 4th May, 1901. Hon. George E. King, Judge Supreme Court Canada, 8th May, 1901. Hon. A. S. Hardy, ex-Premier, Ontario, 14th June, 1901. Sir Thos. Gait, Judge Ontario Supreme Court, 20th June, 1901. Hon. Jos. C. Villeneuve, Senator, Quebec, 27th June, 1901. J. W. Bell, M.P., Addington, 5th July, 1901. Hon. George W. Allan, Senator, Ontario, 24th July, 1901. Hon. Chas. E. Gill, Judge Supreme Court, Quebec, 16th September, 1901. Hon. N. Clarke Wallace, M.P., 8th October, 1901. N. Flood Davin, ex-M.P., 18th October, 1901. J. McMillan, ex-M.P., 31st October, 1901. Geo. F. Orton, ex-M.P., 14th November, 1901. Hon. Mr. Justice Gwyiine, Supreme Court of Canada, 7th January, 1902. " R. R. -
Hastings Law News Vol.21 No.6 UC Hastings College of the Law
University of California, Hastings College of the Law UC Hastings Scholarship Repository Hastings Law News UC Hastings Archives and History 4-14-1988 Hastings Law News Vol.21 No.6 UC Hastings College of the Law Follow this and additional works at: http://repository.uchastings.edu/hln Recommended Citation UC Hastings College of the Law, "Hastings Law News Vol.21 No.6" (1988). Hastings Law News. Book 158. http://repository.uchastings.edu/hln/158 This Book is brought to you for free and open access by the UC Hastings Archives and History at UC Hastings Scholarship Repository. It has been accepted for inclusion in Hastings Law News by an authorized administrator of UC Hastings Scholarship Repository. For more information, please contact [email protected]. Gym fee approved FORUM FEATURES NEWS D.C. Regents should Goren WordPerfect 5.0 Prof. Joseph Sweeney supervise Board of to rave review joins 65 Club elected ASH Directors president .see page 8. .. see .see page 4. 1 "1 1111111111111111111111111111111111111111 II by Chandra K Slack Staff Writer Students went to the polls last week to elect a new set of ASH executive officers for next year. ASH Treasurer Leora Hastings Law News Goren won the hotly contested San FrancLSco, Caltforma April 1-1 , 1988 Volume 21 , :Yo. 6 race for ASH President. Goren ran on a slate with Irma Cor- dova, who ran for Vice Presi- dent, and Phyllis Bursh, a candidate for Treasurer. The slate won a decisive victory in the April 7 election on a plat- KGO Building, West Block form calling for increased stu- dent involvement in decisions at Hastings. -
Journals of the Legislative Assmbly of the Province of Ontario, 1944, Being the First Session Of
JOURNALS OF THE Legislative Assembly OF THE PROVINCE OF ONTARIO From the 22nd February to the 6th April 1944 Both Days Inclusive IN THE EIGHTH YEAR OF THE REIGN OF OUR SOVEREIGN LORD KING GEORGE VI BEING THE First Session of tKe Twenty-First Legislature SESSION 1944 PRINTED BY ORDER OF THE LEGISLATIVE ASSEMBLY VOL. LXXVIII ONTARIO TORONTO Printed and Published by T. E. Bowman, Printer to the King's Most Excellent Majesty 1944 INDEX To the Seventy-Eighth Volume Journals of the Legislative Assembly, Ontario 8 GEORGE VI, 1944 FIRST SESSION TWENTY-FIRST LEGISLATURE February 22nd April 6th,- 1944 POWER AND PAPER COMPANY, LIMITED, MORATORIUM ABITIBIACT, 1944: Bill (No. 36) introduced, 20. 2nd Reading, 22. House in Committee, 24. 3rd Reading, 26. Royal Assent, 156. (8 George VI, c. 1.) ACCOUNTS, PUBLIC: For year ending March 31st, 1943, tabled and referred to Committee on Public Accounts, 16. (Sessional Paper No. 1.) ACTIVE SERVICE ELECTION ACT: Committee appointed to consider revision of, 127. ACTIVE SERVICE FORCES: 1 . Motion asking for increase in clothing allowance to, ruled out of order, 137. 2. See also Armed Forces. ACTIVE SERVICE MORATORIUM ACT, 1943, THE: Bill (No. 60) to amend, introduced, 71. 2nd Reading, 78. House in Committee, 80. 3rd Reading, 84. Royal Assent, 156. (8 George VI, c. 4.) AFFIDAVITS ACT, THE COMMISSIONERS FOR TAKING; Bill (No. 48) to amend, introduced, 42. 2nd Reading, 45. House in Com- mittee and amended, 47. 3rd Reading, 49. Royal Assent, 53. .(8 George VI, c. 12.) AGRICULTURAL ACTIVITIES: Referred to in Speech from Throne, 8. [Hi] iv INDEX 1944 AGRICULTURAL COLLEGE, THE ONTARIO: Release of, from war activities, forecast in Speech from Throne, 8. -
THE REPRESENTATION ACT, 1924 9.—Table Showing Application
THE REPRESENTATION ACT, 1924 73 9.—Table showing Application of Section 51, Subsection 4, of British North America Act, to Representation of Ontario and Nova- Scotia. Ratio of Desrease, Proportion which popula decrease in greater, tion of each province bears Decrease in proportion proportion equal to or Provinces. to the total population of from 1911 to less than one- Canada. from 1911 1921 to twentieth of to 1921. proportion proportion 1911. 1921. in 1911. in 1911. Ontario •35069 •33380 •01689 •0481 less. Nova Scotia •06831 •05960 •00871 •1275 greater. The Representation Act, 1924.—As a result of the census of 1921, a Bill for readjusting the representation in the House of Commons was first introduced in 1923, but was not passed until the close of the 1924 session. This Bill provided for a representation in the fifteenth Parliament of 245 members, taking away 2 members from Nova Scotia (14 instead of 16), and raising the representation of Manitoba from 15 to 17, of Saskatchewan from 16 to 21, of Alberta from 12 to 16, and of British Columbia from 13 to 14, the representation of the rest of the provinces and of the Yukon Territory remaining unaffected. In the re-allotment of seats among the provinces and the total increase of ten members, considerable changes in the boundaries of constituencies have been effected. A summary of these alterations is appended. Prince Edward Island.—No change. Nova Scotia.—The constituencies of Hants and King's are united (Hants- King's); Shelburne and Queen's a're divided, the former being added to Yarmouth and the latter to Lunenburg (Queen's-Lunenburg and Shelburne-Yarmouth); South Cape Breton and Richmond, which formerly elected two members are created separate constituencies, each to return one member (Cape Breton South and Rich mond-West Cape Breton).