Bacterial Long-Chain Polyunsaturated Fatty Acids: Their Biosynthetic Genes, Functions, and Practical Use
Total Page:16
File Type:pdf, Size:1020Kb
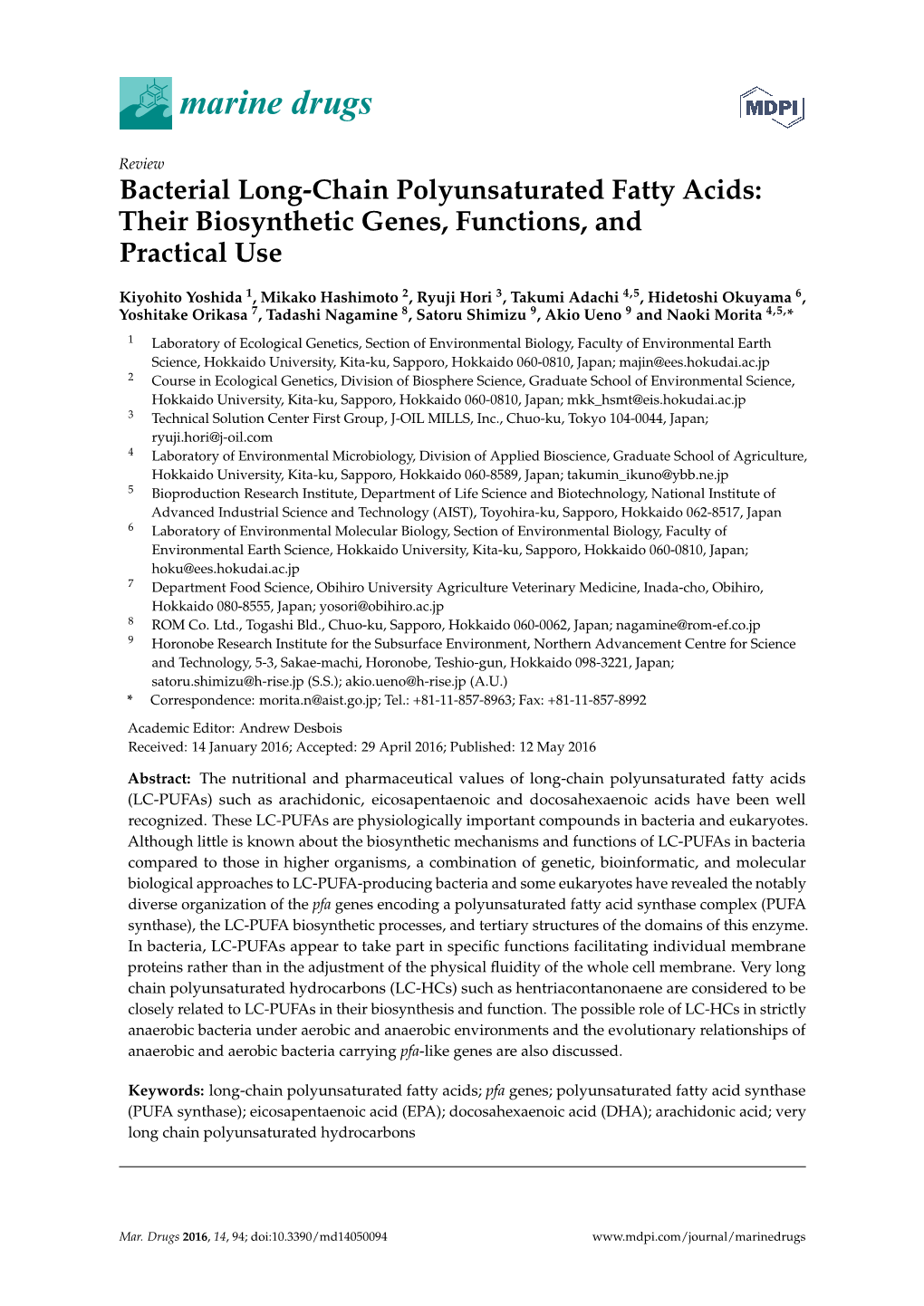
Load more
Recommended publications
-
OMICS Driven Microbial Ecology Hunt for a Core Microbiome
Environmental Microbiology, Volume 14, Issue 1, January 2012 VOLUME 14 NUMBER 1 JANUARY 2012 www.env-micro.com ISSN 1462-2912 Contents environmental microbiology Correspondence 140 Microbial rhodopsins on leaf surfaces of terrestrial plants N. Atamna-Ismaeel, O. M. Finkel, F. Glaser, I. Sharon, R. Schneider, 1 Omics for understanding microbial functional dynamics A. F. Post, J. L. Spudich, C. von Mering, J. A. Vorholt, D. Iluz, O. Béjà & J. K. Jansson, J. D. Neufeld, M. A. Moran & J. A. Gilbert S. Belkin 147 Photoautotrophic symbiont and geography are major factors affecting highly Minireviews structured and diverse bacterial communities in the lichen microbiome 4 Beyond the Venn diagram: the hunt for a core microbiome B. P. Hodkinson, N. R. Gottel, C. W. Schadt & F. Lutzoni A. Shade & J. Handelsman 162 Phosphate transporters in marine phytoplankton and their viruses: environmental cross-domain commonalities in viral-host gene exchanges 13 Targeted metagenomics: a high-resolution metagenomics approach for specifi c A. Monier, R. M. Welsh, C. Gentemann, G. Weinstock, E. Sodergren, gene clusters in complex microbial communities E. V. Armbrust, J. A. Eisen & A. Z. Worden H. Suenaga 177 Complete genome of Candidatus Chloracidobacterium thermophilum, a chlorophyll-based photoheterotroph belonging to the phylum Acidobacteria Research articles A. M. Garcia Costas, Z. Liu, L. P. Tomsho, S. C. Schuster, D. M. Ward & 23 Microbial metatranscriptomics in a permanent marine oxygen minimum zone D. A. Bryant F. J. Stewart, O. Ulloa & E. F. DeLong 191 Transcriptional responses of surface water marine microbial assemblages 41 Genome content of uncultivated marine Roseobacters in the surface ocean to deep-sea water amendment microbiology H. -
Life in the Cold Biosphere: the Ecology of Psychrophile
Life in the cold biosphere: The ecology of psychrophile communities, genomes, and genes Jeff Shovlowsky Bowman A dissertation submitted in partial fulfillment of the requirements for the degree of Doctor of Philosophy University of Washington 2014 Reading Committee: Jody W. Deming, Chair John A. Baross Virginia E. Armbrust Program Authorized to Offer Degree: School of Oceanography i © Copyright 2014 Jeff Shovlowsky Bowman ii Statement of Work This thesis includes previously published and submitted work (Chapters 2−4, Appendix 1). The concept for Chapter 3 and Appendix 1 came from a proposal by JWD to NSF PLR (0908724). The remaining chapters and appendices were conceived and designed by JSB. JSB performed the analysis and writing for all chapters with guidance and editing from JWD and co- authors as listed in the citation for each chapter (see individual chapters). iii Acknowledgements First and foremost I would like to thank Jody Deming for her patience and guidance through the many ups and downs of this dissertation, and all the opportunities for fieldwork and collaboration. The members of my committee, Drs. John Baross, Ginger Armbrust, Bob Morris, Seelye Martin, Julian Sachs, and Dale Winebrenner provided valuable additional guidance. The fieldwork described in Chapters 2, 3, and 4, and Appendices 1 and 2 would not have been possible without the help of dedicated guides and support staff. In particular I would like to thank Nok Asker and Lewis Brower for giving me a sample of their vast knowledge of sea ice and the polar environment, and the crew of the icebreaker Oden for a safe and fascinating voyage to the North Pole. -
Exploiting the Natural Products of Novel Myxobacteria: Phylogenetic and Fatty Acid Perspectives and Bioactive Compound Discovery
Exploiting the natural products of novel myxobacteria: Phylogenetic and fatty acid perspectives and bioactive compound discovery Dissertation zur Erlangung des Grades des Doktors der Naturwissenschaften (Dr. rer. nat.) der Naturwissenschaftlich-Technischen Fakultät III Chemie, Pharmazie, Bio- und Werkstoffwissenschaften der Universität des Saarlandes von Ronald O. Garcia Saarbrücken 2011 Tag des Kolloquiums: 12 August, 2011 Dekan: Univ.-Prof. Dr. Wilhelm F. Maier Berichterstatter: Prof. Dr. Rolf Müller Priv.-Doz. Dr. Marc Stadler Vorsitz: Prof. Dr. Manfred J. Schmitt Akad. Mitarbeiterin: Frau Dr. Kerstin M. Ewen Acknowledgements I sincerely and gratefully thank the following for making my studies possible. Prof. Dr. Rolf Müller, my wonderful adviser, for the trust and giving me the opportunity to work in his laboratory. I am very grateful for the guidance and staunch support during my entire course of my studies. Prof. Dr. Helge Bode, as my second adviser, for his supervision in the laboratory and inspiration. The Helmholtz Zentrum für Infektionsforschung (Helmholtz Centre for Infection Research) and Universität des Saarlandes for funding my PhD study and travel costs for many international conferences. Bundesministerium für Bildung und Forschung (BMBF) and Deutsche Forschungsgemeinschaft (DFG) for the project grants. Dr. Marc Stadler and the staff of InterMed Drug Discovery for their supportive cooperation in PUFA-related projects. Prof. Dr. Irineo J. Dogma Jr. and Prof. Edward Quinto for all their support, motivation, and encouragement for pursuing a PhD. Dr. Alberto Plaza for his excellent advice in compound isolation and Mr. Dominik Pistorius for performing GC-MS measurements of the fatty acids. Dr. Kira J. Wiessman for the inspiration on scientific writing and Dr. -
Appendix 1. Validly Published Names, Conserved and Rejected Names, And
Appendix 1. Validly published names, conserved and rejected names, and taxonomic opinions cited in the International Journal of Systematic and Evolutionary Microbiology since publication of Volume 2 of the Second Edition of the Systematics* JEAN P. EUZÉBY New phyla Alteromonadales Bowman and McMeekin 2005, 2235VP – Valid publication: Validation List no. 106 – Effective publication: Names above the rank of class are not covered by the Rules of Bowman and McMeekin (2005) the Bacteriological Code (1990 Revision), and the names of phyla are not to be regarded as having been validly published. These Anaerolineales Yamada et al. 2006, 1338VP names are listed for completeness. Bdellovibrionales Garrity et al. 2006, 1VP – Valid publication: Lentisphaerae Cho et al. 2004 – Valid publication: Validation List Validation List no. 107 – Effective publication: Garrity et al. no. 98 – Effective publication: J.C. Cho et al. (2004) (2005xxxvi) Proteobacteria Garrity et al. 2005 – Valid publication: Validation Burkholderiales Garrity et al. 2006, 1VP – Valid publication: Vali- List no. 106 – Effective publication: Garrity et al. (2005i) dation List no. 107 – Effective publication: Garrity et al. (2005xxiii) New classes Caldilineales Yamada et al. 2006, 1339VP VP Alphaproteobacteria Garrity et al. 2006, 1 – Valid publication: Campylobacterales Garrity et al. 2006, 1VP – Valid publication: Validation List no. 107 – Effective publication: Garrity et al. Validation List no. 107 – Effective publication: Garrity et al. (2005xv) (2005xxxixi) VP Anaerolineae Yamada et al. 2006, 1336 Cardiobacteriales Garrity et al. 2005, 2235VP – Valid publica- Betaproteobacteria Garrity et al. 2006, 1VP – Valid publication: tion: Validation List no. 106 – Effective publication: Garrity Validation List no. 107 – Effective publication: Garrity et al. -
Contents Topic 1. Introduction to Microbiology. the Subject and Tasks
Contents Topic 1. Introduction to microbiology. The subject and tasks of microbiology. A short historical essay………………………………………………………………5 Topic 2. Systematics and nomenclature of microorganisms……………………. 10 Topic 3. General characteristics of prokaryotic cells. Gram’s method ………...45 Topic 4. Principles of health protection and safety rules in the microbiological laboratory. Design, equipment, and working regimen of a microbiological laboratory………………………………………………………………………….162 Topic 5. Physiology of bacteria, fungi, viruses, mycoplasmas, rickettsia……...185 TOPIC 1. INTRODUCTION TO MICROBIOLOGY. THE SUBJECT AND TASKS OF MICROBIOLOGY. A SHORT HISTORICAL ESSAY. Contents 1. Subject, tasks and achievements of modern microbiology. 2. The role of microorganisms in human life. 3. Differentiation of microbiology in the industry. 4. Communication of microbiology with other sciences. 5. Periods in the development of microbiology. 6. The contribution of domestic scientists in the development of microbiology. 7. The value of microbiology in the system of training veterinarians. 8. Methods of studying microorganisms. Microbiology is a science, which study most shallow living creatures - microorganisms. Before inventing of microscope humanity was in dark about their existence. But during the centuries people could make use of processes vital activity of microbes for its needs. They could prepare a koumiss, alcohol, wine, vinegar, bread, and other products. During many centuries the nature of fermentations remained incomprehensible. Microbiology learns morphology, physiology, genetics and microorganisms systematization, their ecology and the other life forms. Specific Classes of Microorganisms Algae Protozoa Fungi (yeasts and molds) Bacteria Rickettsiae Viruses Prions The Microorganisms are extraordinarily widely spread in nature. They literally ubiquitous forward us from birth to our death. Daily, hourly we eat up thousands and thousands of microbes together with air, water, food. -
Molecular Studies on the Role of Bacteria in a Marine Algal Disease
Molecular Studies on the Role of Bacteria in a Marine Algal Disease Neil Daniel Fernandes A thesis in fulfillment of the requirements for the degree of Doctor of Philosophy School of Biotechnology and Biomolecular Sciences Faculty of Science The University of New South Wales Sydney, Australia April, 2011 PLEASE TYPE THE UNIVERSITY OF NEW SOUTH WALES Thesis/Dissertation Sheet Surname or Family name Femandes First name Neil Other name/s Daniel Abbrev1at1on for degree as given in the Un1versity calendar PhD School School of Biotechnology and Biomolecular Sciences Faculty Science Title. Molecular Studies on the Role of Bacteria in a Marine Algal Disease Abstract 350 words maximum: {PLEASE TYPE) Disease is increasingly viewed as a major factor in marine ecology and its impact is expected to increase with environmental change such as global wanning. This thesis focuses on understanding a "bleaching" disease. which affects the temperate-water red macroalga Delisea pulchra, particularly in summer when sea water temperatme.., are elevated. The bleaching disease could be reproduced in vitro by a combination of increased temperature and the presence of bacterial pathogens. which are phylogenetically affiliated with the genera Microbulhljer. Alteromonas, Cellulophaga and the marine Roseobacter lineage. Since only a fraction of environmental bacteria are cultivable by standard laboratory techniques, a culture-independent metagenomics approach was used ll' characterize the ph) logenetic and functional shifts induced by bleaching in the surface-associated. bacterial community. Phylogenetic shifts mainly reflected relative changes in the genera Tha/assomonas. Thioc/a\'tl and Parvularcula. while changes in gene composition were mainly due to functions associated with transcriptional regulation. -
Genome-Based Taxonomic Classification Of
ORIGINAL RESEARCH published: 20 December 2016 doi: 10.3389/fmicb.2016.02003 Genome-Based Taxonomic Classification of Bacteroidetes Richard L. Hahnke 1 †, Jan P. Meier-Kolthoff 1 †, Marina García-López 1, Supratim Mukherjee 2, Marcel Huntemann 2, Natalia N. Ivanova 2, Tanja Woyke 2, Nikos C. Kyrpides 2, 3, Hans-Peter Klenk 4 and Markus Göker 1* 1 Department of Microorganisms, Leibniz Institute DSMZ–German Collection of Microorganisms and Cell Cultures, Braunschweig, Germany, 2 Department of Energy Joint Genome Institute (DOE JGI), Walnut Creek, CA, USA, 3 Department of Biological Sciences, Faculty of Science, King Abdulaziz University, Jeddah, Saudi Arabia, 4 School of Biology, Newcastle University, Newcastle upon Tyne, UK The bacterial phylum Bacteroidetes, characterized by a distinct gliding motility, occurs in a broad variety of ecosystems, habitats, life styles, and physiologies. Accordingly, taxonomic classification of the phylum, based on a limited number of features, proved difficult and controversial in the past, for example, when decisions were based on unresolved phylogenetic trees of the 16S rRNA gene sequence. Here we use a large collection of type-strain genomes from Bacteroidetes and closely related phyla for Edited by: assessing their taxonomy based on the principles of phylogenetic classification and Martin G. Klotz, Queens College, City University of trees inferred from genome-scale data. No significant conflict between 16S rRNA gene New York, USA and whole-genome phylogenetic analysis is found, whereas many but not all of the Reviewed by: involved taxa are supported as monophyletic groups, particularly in the genome-scale Eddie Cytryn, trees. Phenotypic and phylogenomic features support the separation of Balneolaceae Agricultural Research Organization, Israel as new phylum Balneolaeota from Rhodothermaeota and of Saprospiraceae as new John Phillip Bowman, class Saprospiria from Chitinophagia. -
Diversity of Marine Gliding Bacteria in Thailand and Their Cytotoxicity
Electronic Journal of Biotechnology ISSN: 0717-3458 Vol.12 No.3, Issue of July 15, 2009 © 2009 by Pontificia Universidad Católica de Valparaíso -- Chile Received October 7, 2008 / Accepted March 29, 2009 DOI: 10.2225/vol12-issue3-fulltext-13 RESEARCH ARTICLE Diversity of marine gliding bacteria in Thailand and their cytotoxicity Yutthapong Sangnoi Department of Industrial Biotechnology Faculty of Agro-Industry Prince of Songkla University Hat Yai, Songkhla 90112, Thailand Pornpoj Srisukchayakul Thailand Institute of Scientific and Technological Research 35 Moo 3, Technopolis, Khlong 5, Khlong Luang Pathum Thani 12120, Thailand Vullapa Arunpairojana Thailand Institute of Scientific and Technological Research 35 Moo 3, Technopolis, Khlong 5, Khlong Luang Pathum Thani 12120, Thailand Akkharawit Kanjana-Opas* Department of Industrial Biotechnology Faculty of Agro-Industry Prince of Songkla University Hat Yai, Songkhla 90112, Thailand Tel: 66 74286373 Fax: 66 74212889 E-mail: [email protected] Financial support: Thailand Research Fund (MRG4880153) and a Biodiversity Research and Training Grant (BRTR_149011). Scholarship for YS from the Graduate School, Prince of Songkla University. Keywords: Aureispira marina, Aureispira maritime, Fulvivirga kasyanovii, human cell lines, Rapidithrix thailandica, Tenacibaculum mesophilum. Abbreviations: CFB: Cytophaga-Flavobacterium-Bacteriodes HeLa: cervical cancer HT-29: colon cancer KB: oral cancer MCF-7: breast adenocarcinoma PCR: polymerase chain reaction SK: skim milk medium SRB: sulphorodamine B Eighty-four marine gliding bacteria were isolated from thailandica, Aureispira marina and Aureispira maritima specimens collected in the Gulf of Thailand and the respectively. The isolates were cultivated in four Andaman Sea. All exhibited gliding motility and swarm different cultivation media (Vy/2, RL 1, CY and SK) colonies on cultivation plates and they were purified by and the crude extracts were submitted to screen subculturing and micromanipulator techniques. -
The Influence of Invasive Jellyfish Blooms on The
Biol Invasions DOI 10.1007/s10530-014-0810-2 MOLECULAR TOOLS The influence of invasive jellyfish blooms on the aquatic microbiome in a coastal lagoon (Varano, SE Italy) detected by an Illumina-based deep sequencing strategy Caterina Manzari • Bruno Fosso • Marinella Marzano • Anita Annese • Rosa Caprioli • Anna Maria D’Erchia • Carmela Gissi • Marianna Intranuovo • Ernesto Picardi • Monica Santamaria • Simonetta Scorrano • Giuseppe Sgaramella • Loredana Stabili • Stefano Piraino • Graziano Pesole Received: 14 November 2013 / Accepted: 31 October 2014 Ó The Author(s) 2014. This article is published with open access at Springerlink.com Abstract The rapid expansion of multicellular microbial taxonomic and functional diversity. Here native and alien species outbreaks in aquatic and we used an effective and highly sensitive experimental terrestrial ecosystems (bioinvasions) may produce strategy, bypassing the efficiency bottleneck of the significant impacts on bacterial community dynamics traditional bacterial isolation and culturing method, to and nutrient pathways with major ecological implica- identify changes of the planktonic microbial commu- tions. In aquatic ecosystems, bioinvasions may cause nity inhabiting a marine coastal lagoon (Varano, adverse effects on the water quality resulting from Adriatic Sea) under the influence of an outbreak- changes in biological, chemical and physical proper- forming alien jellyfish species. Water samples were ties linked to significant transformations of the collected from two areas that differed in their level of confinement inside in the lagoon and jellyfish densities (W, up to 12.4 medusae m-3; E, up to 0.03 medusae Caterina Manzari, Bruno Fosso and Marinella Marzano have -3 contributed equally to this work. m ) to conduct a snapshot microbiome analysis by a metagenomic approach. -
Bacterial Community Dynamics Are Linked to Patterns of Coral Heat Tolerance
ARTICLE Received 28 Aug 2016 | Accepted 2 Dec 2016 | Published 10 Feb 2017 DOI: 10.1038/ncomms14213 OPEN Bacterial community dynamics are linked to patterns of coral heat tolerance Maren Ziegler1, Francois O. Seneca2, Lauren K. Yum1, Stephen R. Palumbi2 & Christian R. Voolstra1 Ocean warming threatens corals and the coral reef ecosystem. Nevertheless, corals can be adapted to their thermal environment and inherit heat tolerance across generations. In addition, the diverse microbes that associate with corals have the capacity for more rapid change, potentially aiding the adaptation of long-lived corals. Here, we show that the microbiome of reef corals is different across thermally variable habitats and changes over time when corals are reciprocally transplanted. Exposing these corals to thermal bleaching conditions changes the microbiome for heat-sensitive corals, but not for heat-tolerant corals growing in habitats with natural high heat extremes. Importantly, particular bacterial taxa predict the coral host response in a short-term heat stress experiment. Such associations could result from parallel responses of the coral and the microbial community to living at high natural temperatures. A competing hypothesis is that the microbial community and coral heat tolerance are causally linked. 1 Red Sea Research Center, Division of Biological and Environmental Science and Engineering (BESE), King Abdullah University of Science and Technology (KAUST), Building 2, Thuwal 23955-6900, Saudi Arabia. 2 Hopkins Marine Station, Stanford University, 120 Ocean View Blvd, Pacific Grove, California 93950, USA. Correspondence and requests for materials should be addressed to S.R.P. (email: [email protected]) or to C.R.V. (email: [email protected]). -
Corals at the Extreme: Partitioning the Response of Coral Holobionts to Marginal Habitats
Corals at the extreme: partitioning the response of coral holobionts to marginal habitats Bethan Greenwood A thesis submitted for the degree of Doctor of Philosophy in Marine Biology School of Life Sciences University of Essex October 2020 Abstract While coral reefs worldwide are threatened by unprecedented environmental change, some reef-building corals can already be found living under extreme conditions within marginal habitats. Learning how corals can survive high temperature fluctuations and multiple other stressors experienced in mangroves, relative to typical reefs, is a key step in understanding the adaptive capacity of reef-building corals to future environmental change. The role of the coral host, symbiotic algae, and diverse microbiota, and how these components of the holobiont interact to define the adaptive capacity of reef-building corals requires further exploration. In this thesis, the thermal tolerance limits of conspecific corals from a mangrove versus a reef habitat were tested in a 20-day heat-ramping experiment. Heating corals beyond their regional thermal maxima caused severe decreases in productivity, irrespective of which habitat the coral came from, but corals from the mangrove habitat suffered less thermally induced bleaching. Amplicon sequencing coral holobionts from reef and mangrove habitats in Indonesia and the Seychelles revealed significant habitat-dependent differences in coral microbiome compositions. A potentially novel coral-bacteria symbiosis between a mangrove-dwelling merulinid coral and an unclassified spirochaete, which accounted for 47% of the coral’s bacterial community, was also uncovered, though its role in the holobiont remains unknown. Reciprocal translocations of corals between reef and mangrove habitats resulted in rapid reorganisation of coral-associated bacterial communities. -
Diversity of Marine Gliding Bacteria in Thailand and Their Cytotoxicity
Electronic Journal of Biotechnology ISSN: 0717-3458 Vol.12 No.3, Issue of July 15, 2009 © 2009 by Pontificia Universidad Católica de Valparaíso -- Chile Received October 7, 2008 / Accepted March 29, 2009 DOI: 10.2225/vol12-issue3-fulltext-13 RESEARCH ARTICLE Diversity of marine gliding bacteria in Thailand and their cytotoxicity Yutthapong Sangnoi Department of Industrial Biotechnology Faculty of Agro-Industry Prince of Songkla University Hat Yai, Songkhla 90112, Thailand Pornpoj Srisukchayakul Thailand Institute of Scientific and Technological Research 35 Moo 3, Technopolis, Khlong 5, Khlong Luang Pathum Thani 12120, Thailand Vullapa Arunpairojana Thailand Institute of Scientific and Technological Research 35 Moo 3, Technopolis, Khlong 5, Khlong Luang Pathum Thani 12120, Thailand Akkharawit Kanjana-Opas* Department of Industrial Biotechnology Faculty of Agro-Industry Prince of Songkla University Hat Yai, Songkhla 90112, Thailand Tel: 66 74286373 Fax: 66 74212889 E-mail: [email protected] Financial support: Thailand Research Fund (MRG4880153) and a Biodiversity Research and Training Grant (BRTR_149011). Scholarship for YS from the Graduate School, Prince of Songkla University. Keywords: Aureispira marina, Aureispira maritime, Fulvivirga kasyanovii, human cell lines, Rapidithrix thailandica, Tenacibaculum mesophilum. Abbreviations: CFB: Cytophaga-Flavobacterium-Bacteriodes HeLa: cervical cancer HT-29: colon cancer KB: oral cancer MCF-7: breast adenocarcinoma PCR: polymerase chain reaction SK: skim milk medium SRB: sulphorodamine B Eighty-four marine gliding bacteria were isolated from thailandica, Aureispira marina and Aureispira maritima specimens collected in the Gulf of Thailand and the respectively. The isolates were cultivated in four Andaman Sea. All exhibited gliding motility and swarm different cultivation media (Vy/2, RL 1, CY and SK) colonies on cultivation plates and they were purified by and the crude extracts were submitted to screen subculturing and micromanipulator techniques.