Evaluating Tools Used to Estimate and Manage Browse Available to Wintering Moose on the Copper River Delta, Alaska
Total Page:16
File Type:pdf, Size:1020Kb
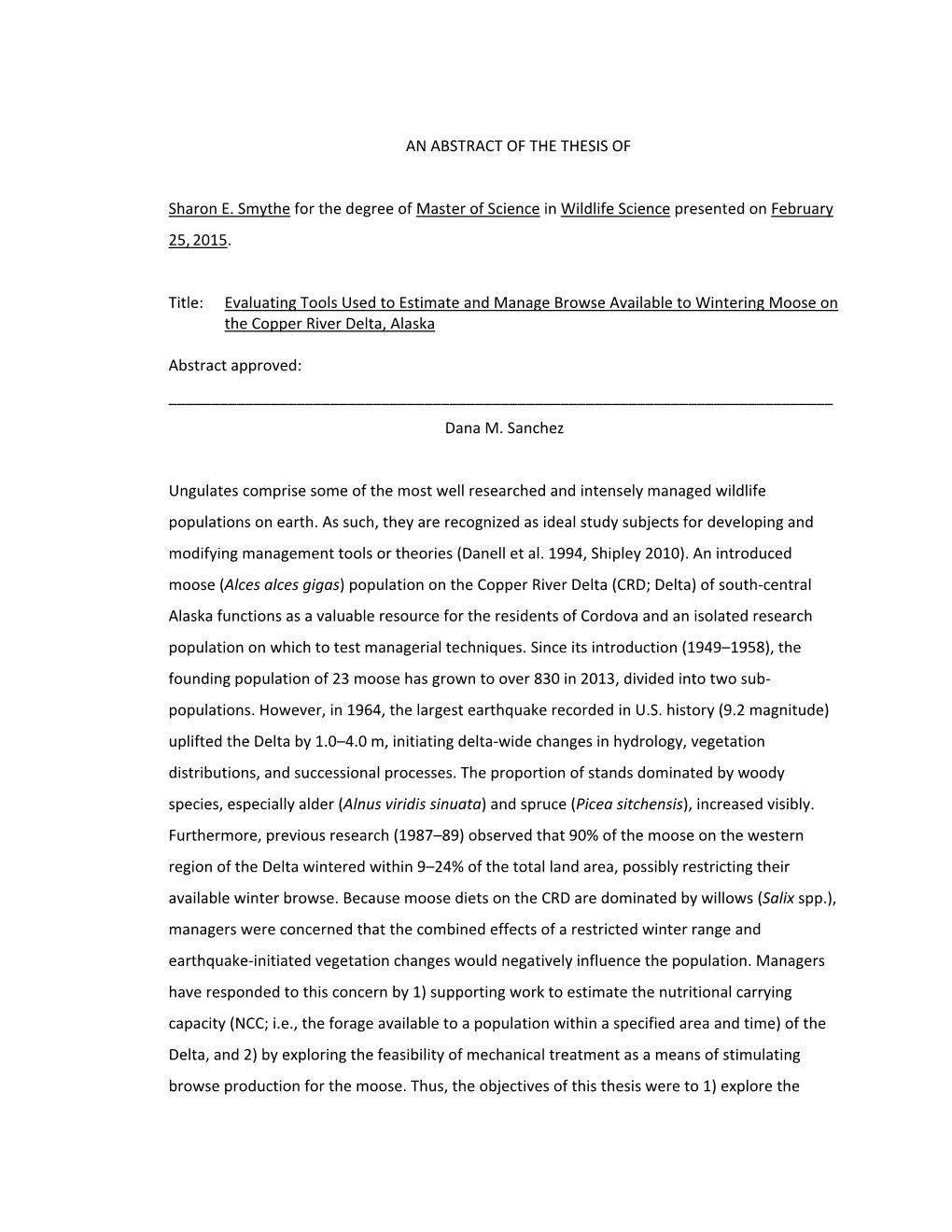
Load more
Recommended publications
-
The Ecology and Management of Moose in North America
THE ECOLOGY AND MANAGEMENT OF MOOSE IN NORTH AMERICA Douglas H. PIMLOTT Department of Lands and Forests, Maple, Ontario, Canada Concepts of the status, productivity and management of North American moose (Alces alces) have changed greatly during the past decade. The rapidity of the change is illustrated by the published record. TUFTS (1951) questioned, « Is the moose headed for extinc tion ? » and discussed the then current belief that moose populations had seriously declined across much of the continent. Five years later, PETERSON (1955: 217) stated, « It appears almost inevitable that the days of unlimited hunting for moose must soon pass from most of North America. » He also suggested (1955 : 216) that a kill of 12 to 25 per cent of the adult population is the highest that would permit the maintenance of the breeding population. Four years later, I showed (PIMLOTT, 1959a) that moose in Newfoundland could sustain a kill of twice the magnitude suggested by Peterson. I also suggested (PIMLOTT, 1959b) that the North American moose kill could be very greatly increased-in spite of progressive liberalization of hunting regulations over much of Canada and a marked increase in annual kill. It is not realistic to assume that the status of the species has changed, within the decade, from threatened extinction to annual harvests of approximately 40,000 and potential harvests of two to three times that number. Although moose populations have increased in some areas since 1950, there is little doubt that the changed think ing about moose management is more the result of the increase in knowledge than of any other factor. -
Moose – Preparing for the Future an Action Plan for the Moose
Wildlife Stewardship SERIES IV NOVEMBER 2009 Moose – Preparing for the Future An Action Plan for the Moose 0$&.( ) 1= 2 ,( 1 0 2 , 2 7 8 $ , 1 7 & $ 2 , 1 6 6 6 $ In cooperation with the Ministry of Environment TM 287),77(56 Workshop Recommendations 1. Communicating the habitat needs of moose to other resource ministries and users through land-use plans will ensure a holistic approach to wildlife management. 2. Adjusting moose management in response to increased hunter access due to logging is important for sustainability and balanced age-class structures. 3. A commitment to the North American Wildlife Conservation Model would improve moose management in BC. The model outlines management principles and stipulates that law and science should be the foundation for wildlife management. 4. Improved harvest data collection from First Nations and resident recreational hunters would enhance the foundation guiding wildlife management decisions. 5. Including spike-fork moose in the annual allowable harvest (AAH) will improve moose management in BC. 6. Hunting regulations should reflect the increased percentage of yearling spike- fork moose. 7. There are other sources of funding for population surveys available to the Ministry of Environment. Guide outfitters can play an important role as the “on the ground” surveyors of animal populations. 8. Collaborative efforts between the Ministry of Environment, Ministry of Transportation and Infrastructure, and Insurance Corporation of British Columbia (ICBC) to improve education, signage, and fencing (with overpasses or underpasses) at high collision locations would reduce vehicle/moose collisions. 9. Working collaboratively with the University of Northern British Columbia (UNBC), the Ministry of Environment and Ministry of Transportation and Infrastructure can encourage railway companies to take preventative measures to reduce train/moose collisions. -
Moose (Alces Alces) Browse Enhancement and Sustainable Forestry As a Rural Development Tool in the Sub-Arctic Boreal Forest Region of Alaska
Moose (Alces alces) browse enhancement and sustainable forestry as a rural development tool in the sub-Arctic boreal forest region of Alaska Item Type Other Authors Cain, Bruce David Download date 08/10/2021 00:41:31 Link to Item http://hdl.handle.net/11122/9759 MOOSE (Alces alces) BROWSE ENHANCEMENT AND SUSTAINABLE FORESTRY AS A RURAL DEVELOPMENT TOOL IN THE SUB-ARCTIC BOREAL FOREST REGION OF ALASKA AN APPLIED COMMUNITY DEVELOPMENT PROJECT Presented to the Faculty of the University of Alaska Fairbanks in Partial Fulfillment of the Requirements for the Degree of MASTER OF ARTS RURAL DEVELOPMENT By Bruce David Cain, B.A., A.A. Fairbanks, Alaska May 2014 Abstract This project studies indigenous and western moose browse management issues in the sub-arctic boreal forest and how this topic relates to rural development. Chapter one explains the methodology of the project. Chapter two describes how moose browse and biomass management support rural development and investigates productivity potential of combining moose browse management with sustainable forestry and biomass production. Chapter three investigates landscape and habitat management principles from a customary and traditional practice versus a scientific approach. It looks at management models in the following territories: Alaska, Canada, Continental US, Mongolia/Russia and Scandinavia. Chapter four investigates indigenous wildlife management systems and other indigenous wildlife policy issues. Chapter five is a selected annotated bibliography. The project has a focus on the Ahtna region of central Alaska and recognizes the implications of these issues for this region. i In Memoriam Walter Charley was a man who inspired many across cultural and technical boundaries. -
Status and Trends of Moose Populations and Hunting Opportunity in the Western United States
STATUS AND TRENDS OF MOOSE POPULATIONS AND HUNTING OPPORTUNITY IN THE WESTERN UNITED STATES M. Steven Nadeau1, Nicholas J. DeCesare2, Douglas G. Brimeyer3, Eric J. Bergman4, Richard B. Harris5, Kent R. Hersey6, Kari K. Huebner7, Patrick E. Matthews8, and Timothy P. Thomas9 1Idaho Department of Fish and Game, 600 S. Walnut, Boise, Idaho 83709, USA; 2Montana Fish, Wildlife and Parks, 3201 Spurgin Road, Missoula, Montana 59804, USA; 3Wyoming Game and Fish Department, Box 67, Jackson, Wyoming 83001, USA; 4Colorado Parks and Wildlife, 317 W. Prospect Avenue, Fort Collins, Colorado 80526, USA; 5Washington Department of Fish and Wildlife, 600 Capital Way North, Olympia, Washington 98504, USA; 6Utah Division of Wildlife Resources, Box 146301, Salt Lake City, Utah 84114, USA; 7Nevada Department of Wildlife, 60 Youth Center Road, Elko, Nevada, 89801; 8Oregon Department of Fish and Wildlife, 65495 Alder Slope Road, Enterprise, Oregon 97828, USA; 9Wyoming Game and Fish Department, Box 6249, Sheridan, Wyoming 82801, USA. ABSTRACT: We review the state of knowledge of moose (Alces alces shirasi) in the western US with respect to the species’ range, population monitoring and management, vegetative associations, licensed hunting opportunity and hunter harvest success, and hypothesized limiting factors. Most moose monitoring programs in this region rely on a mixture of aerial surveys of various formats and hunter harvest statistics. However, given the many challenges of funding and collecting rigorous aerial survey data for small and widespread moose populations, biologists in many western states are currently exploring other potential avenues for future population monitoring. In 2015, a total of 2,263 hunting permits were offered among 6 states, with 1,811 moose harvested and an average success rate per permit-holder of 80%. -
Status and Trends of Moose Populations and Hunting Opportunity in the Western United States
STATUS AND TRENDS OF MOOSE POPULATIONS AND HUNTING OPPORTUNITY IN THE WESTERN UNITED STATES M. Steven Nadeau1, Nicholas J. DeCesare2, Douglas G. Brimeyer3, Eric J. Bergman4, Richard B. Harris5, Kent R. Hersey6, Kari K. Huebner7, Patrick E. Matthews8, and Timothy P. Thomas9 1Idaho Department of Fish and Game, 600 S. Walnut, Boise, Idaho 83709, USA; 2Montana Fish, Wildlife and Parks, 3201 Spurgin Road, Missoula, Montana 59804, USA; 3Wyoming Game and Fish Department, Box 67, Jackson, Wyoming 83001, USA; 4Colorado Parks and Wildlife, 317 W. Prospect Avenue, Fort Collins, Colorado 80526, USA; 5Washington Department of Fish and Wildlife, 600 Capital Way North, Olympia, Washington 98504, USA; 6Utah Division of Wildlife Resources, Box 146301, Salt Lake City, Utah 84114, USA; 7Nevada Department of Wildlife, 60 Youth Center Road, Elko, Nevada, 89801; 8Oregon Department of Fish and Wildlife, 65495 Alder Slope Road, Enterprise, Oregon 97828, USA; 9Wyoming Game and Fish Department, Box 6249, Sheridan, Wyoming 82801, USA. ABSTRACT: We review the state of knowledge of moose (Alces alces shirasi) in the western US with respect to the species’ range, population monitoring and management, vegetative associations, licensed hunting opportunity and hunter harvest success, and hypothesized limiting factors. Most moose monitoring programs in this region rely on a mixture of aerial surveys of various formats and hunter harvest statistics. However, given the many challenges of funding and collecting rigorous aerial survey data for small and widespread moose populations, biologists in many western states are currently exploring other potential avenues for future population monitoring. In 2015, a total of 2,263 hunting permits were offered among 6 states, with 1,811 moose harvested and an average success rate per permit-holder of 80%. -
Moose-Habitat Relationships in the Yaak River Drainage Northwestern Montana
University of Montana ScholarWorks at University of Montana Graduate Student Theses, Dissertations, & Professional Papers Graduate School 1985 Moose-habitat relationships in the Yaak River drainage northwestern Montana Marc R. Matchett The University of Montana Follow this and additional works at: https://scholarworks.umt.edu/etd Let us know how access to this document benefits ou.y Recommended Citation Matchett, Marc R., "Moose-habitat relationships in the Yaak River drainage northwestern Montana" (1985). Graduate Student Theses, Dissertations, & Professional Papers. 7378. https://scholarworks.umt.edu/etd/7378 This Thesis is brought to you for free and open access by the Graduate School at ScholarWorks at University of Montana. It has been accepted for inclusion in Graduate Student Theses, Dissertations, & Professional Papers by an authorized administrator of ScholarWorks at University of Montana. For more information, please contact [email protected]. COPYRIGHT ACT OF 1976 Th is is an unpublished manuscript in which copyright sub s is t s. Any further r eprinting of its contents must be approved BY the author. MANSFIELD L ibrary Un iv er s ity of Montana Date ; 1 ^ 8 5 _______ Reproduced with permission of the copyright owner. Further reproduction prohibited without permission. Reproduced with permission of the copyright owner. Further reproduction prohibited without permission. MOOSE-HABITAT RELATIONSHIPS IN THE ÏAAK RIVER DRAINAGE, NORTHWESTERN MONTANA by Marc R. Hatchett B.S. University of Montana, 1980 Presented in partial fulfillment of the requirements for the degree of Master of Science University of Montana 1985 Approved by; c : - ; / , / / , Chairman, Board1 of/Examinerso f V E x a Dean, Graduate School /Sl/ Ù?/y :>' Date Reproduced with permission of the copyright owner. -
• a Review of Moose Food Habits Studies in North America
[]{]&OO~& c ~IID&~©@ Susitna Joint Venture Document Number Please Return To DOCUMENT CONTROL A REVIEW OF MOOSE FOOD HABITS STUDIES IN NORTH AMERICA • . [va/WilliS/I! can., 101: 195.215 (197;] A REVIE'vV OF MOOSE FOOD HABITS STUDIES IN NORTH AMERICA I J. M. PEEK2 Department of Entomology, Fisheries and Wildlife, University of Minnesota, St. Paul, Minnesota, United States Resume :';i ... .:.: q -.. ::t .-,; ,~~]. Cet article passe en revue 41 etudes portant sur les habitudes alimentaires de ~~. , I'orignal, qont 13 ont ete effectuees dans la cordilliere interieure. 6 en Alaska et 22 .. .' : au Canada, au Minnesota, a I'Isle Royale et dans Ie Maine. Seulement neuf de ces 'C etudes traitent des habitudes alimentair~estivales. alors que seulement quatre de cel ~ ., 0 .. les-d traitent de la pMnologie annuelle des habitudes alimentaires de I'orignal et seule :~ ment deux etudes s'etendentsur plus d'un an. Les variations locales des habitudes ali .. 1."; mentaires sont tres importantes et des gimeralisations concernant les especes pre ~-:; ..; ~ ferees, sans que soit corroboree I'information pour une region donnee, apparaissent ........~ - .. risquees. Unecombinaison des methodes utilisees semble pertinente, car chaque methode '.~.. ., a ses restrictions propres. Bien qu'une vue d'ensemble pour I'Amerique du Nord puisse ;,. .•. '~;"I etre tracee partir de I'information disponible, I'auteur conclut neanmoins que les ;_0' a }", donnees manquent pour comparer, entre differentes regions. les patrons d'utilisation .-\1 ~ annuels, saisonniers de meme qu'en fonction des differents types d'habitats. L'auteur e estime qu'it est essentiel d'evaluer les habitudes alimentaires avant d'apprecier les conditions du milieu et leurs changements, ou, avant d'entreprendre des recherches portant sur la valeur nutritive et la digestibilite des dilferentes especes vegetales concernees. -
Rl~Ed 1T4 Eeata99
askaReso~ rces~!~nrormationSctv l c, U brary BUik1 tn ~ . Sui t<.: ll l 3211 Proviucii.rc Drive TH £ Mo 0 SE 1\nchorage, tuK 9~508-46 1 4 rl~ed 1t4 Eeata99 WILDLIFE LEAFLET 312 ISH AND 'WIL DLIFE SERVICE UNITED STATES DEPARTMENT OF THE INTERIOR -J l UNITED STATES DEP.ARIJ:KENT OF THE INTERIOR •• J. A· Krug, Secretary FISH JU.."D WILDLIFE SERVICE •••••Albert M. nay, Director . l ' ~-] l ] FRONTISPIECE: AKontana Bull ·Photo by-Montana Fish and Game· Co.mmi,.ssio!l l j -1 J washington 25, D. c. October 1949 L r THE MOOSE AND ITS ECOLOGY [ By N. w. Hosley, B:l.ologist, In Charge section of Wildlife Investigations on Public Lands [ Branch of Wildlife Research [ c CONTENTS [ Page Page Introduction . • • • • • • • . 1 Management • . • . • . • . • 37 Distribution and economics • • 2 Census methods. • . • 37 Lite history • . • . • . 4 Protection and control • [ Characteristics affecting. pf kill. 38 management. • • . • . 11 . Range improvement . •. 38 Senses. • . • • . • • • 11 Carrying capacity 39 [ Social habits . • . • 12 : Productivity. 39 Populations • • • • .. • • 12 . Sanctuaries . • . • . 40 Movements • • . 15 stocking. 42 [ Foods and feeding • • • . • 18 Conclusions. • . 42 cover • • . • • . 32 Acknowledgments . 43 Moose-beaver relationships. 33 : Bibliography • . • . 44 Decimating factors. 33 [ • . • [ INTRODUCTION [ The general decline o:r moose populations bas aroused great in terest, and numerous popular articles have appeared on the subject. Since 1929, however, no attempt has been made to bring together the [ information that has been steadily accumulating concerning this 4 important big game animal and its management. With this in mind the author has undertaken the task. L As the moose is an animal difficult to observe, large, and un \ usual in form and action; it is an ideal subject about which to write unnatural history. -
Heterogeneity and Power to Detect Trends in Moose Browse Utilization of Willow Communities
HETEROGENEITY AND POWER TO DETECT TRENDS IN MOOSE BROWSE UTILIZATION OF WILLOW COMMUNITIES Braden O. Burkholder1,4, Nicholas J. DeCesare2, Robert A. Garrott1, and Sylvanna J. Boccadori3 1Fish and Wildlife Ecology and Management Program, Department of Ecology, Montana State University, 310 Lewis Hall, Bozeman, Montana, 59717 USA; 2Montana Fish, Wildlife and Parks, 3201 Spurgin Road, Missoula, Montana, 59804 USA; 3Montana Fish, Wildlife and Parks, 1820 Meadowlark Lane, Butte, Montana, 59701 USA. ABSTRACT: Monitoring of browse utilization of plant communities is consistently recommended as an important component of monitoring moose (Alces alces) populations across regions. We moni- tored winter browse utilization by moose within a willow (Salix spp.) -dominated winter range of Montana in 2008–2010. We sought to improve our understanding of: 1) spatiotemporal hetero- geneity of intensity of moose browsing across the winter range, 2) species-specific selection of willow by moose during winter, and 3) appropriate sample sizes, placement, and stratification of monitoring sites for estimating browse utilization. During 3 consecutive winters we monitored 108–111 transect segments, each 50 m in length, in a systematic distribution across willow communities and assessed the effects of covariates potentially predictive of variation in browsing. Mean annual estimated browse utilization across all segments was 11.5% of sampled twigs in 2008 (95% CI = 9.4 – 13.7%), 8.0% in 2009 (95% CI = 6.2 – 9.8%), and 8.3% in 2010 (95% CI = 6.5 – 10.1%). Modeling of variation in browse utilization revealed positive relationships with the proportion of preferred species (β = 0.44, P = 0.05) and previously browsed willow plants (β = 3.13, P < 0.001), and a negative relationship with willow patch width (β = 0.002, P < 0.001). -
The Biogeography of the Caribou Lungworm, Varestrongylus
Edinburgh Research Explorer The biogeography of the caribou lungworm, Varestrongylus eleguneniensis (Nematoda Citation for published version: Verocai, GG, Hoberg, EP, Simard, M, Beckmen, KB, Musiani, M, Wasser, S, Cuyler, C, Manseau, M, Chaudhry, UN, Kashivakura, CK, Gilleard, JS & Kutz, SJ 2020, 'The biogeography of the caribou lungworm, Varestrongylus eleguneniensis (Nematoda: Protostrongylidae) across northern North America', International Journal for Parasitology: Parasites and Wildlife, vol. 11, pp. 93-102. https://doi.org/10.1016/j.ijppaw.2020.01.001 Digital Object Identifier (DOI): 10.1016/j.ijppaw.2020.01.001 Link: Link to publication record in Edinburgh Research Explorer Document Version: Publisher's PDF, also known as Version of record Published In: International Journal for Parasitology: Parasites and Wildlife General rights Copyright for the publications made accessible via the Edinburgh Research Explorer is retained by the author(s) and / or other copyright owners and it is a condition of accessing these publications that users recognise and abide by the legal requirements associated with these rights. Take down policy The University of Edinburgh has made every reasonable effort to ensure that Edinburgh Research Explorer content complies with UK legislation. If you believe that the public display of this file breaches copyright please contact [email protected] providing details, and we will remove access to the work immediately and investigate your claim. Download date: 28. Sep. 2021 IJP: Parasites and Wildlife 11 (2020) 93–102 Contents lists available at ScienceDirect IJP: Parasites and Wildlife journal homepage: www.elsevier.com/locate/ijppaw The biogeography of the caribou lungworm, Varestrongylus eleguneniensis (Nematoda: Protostrongylidae) across northern North America T ∗ Guilherme G. -
Rural Economy
378.7123 D47 P-94-2 RURAL ECONOMY PROJECT REPORT WAITE MEMORIAL BOOK DEPT. COLLECTION OF AG. AND APPLIED 1994 ECONOMICS BUFORD AVE. - 232 COR UNIVERSITY OF ST. MINNESOTA PAUL MN 55108 U.S.A. TrL.\61 Department of Rural Economy Mori Faculty of Agriculture and Forestry University of Alberta Edmonton, Canada 3-2tP.7i:13 • Economic Effects of Environmental Quality Change on Recreational Hunting in Northern Saskatchewan .K. Morton, W.L. Adamowicz and P.C. Boxall Project Report 94-02 WAITE MEMORIAL BOOK COLLECTION DEPT. OF AG. AND APPLIED ECONOMICS 1994 BUFORD AVE. -232 COB UNIVERSITY OF MINNESOTA ST. PAUL, MN 55108 U.S.A. Alberta, Associate Professor, The authors are Research Assistant, Department of Rural Economy,,University of Forestry Canada, Department of Rural Economy, University of Alberta, and Non-timber Valuation Economist, Edmonton. ••• ACKNOWLEDGEMENTS We would like to thank the following organizations for their help in the design and implementation of this project: Forestry Canada, Mistik Management Ltd., Terrestrial and Aquatic Environmental Management, Saskatchewan Environment and Resource Management, Saskatchewan Wildlife Branch, and Dalpe's Specialized Services Ltd. We would also like to thank the following organizations for their support in this project by providing prizes for survey respondents: Forestry Canada, National Firearms Association, Canadian Outdoor Publications Inc., Ducks Unlimited, Saskatchewan Wildlife Federation, Millar Western and Trout Unlimited. ABSTRACT This study was undertaken to provide some of the social values for the non-timber component of the Millar Western-NorSask Forest Management Licence Agreement. This study estimates the changes in the value of a recreational hunting experience as one, or a combination of several, of the following items change in the forest environment: i) road access; ii) game populations; iii) congestion; and iv) travel distance. -
Download The
THE LIFE OF A NAVVY: A STUDY OF THE RELATIONSHIP BETWEEN ETHNICITY AND STATUS WITHIN RAILWAY WORK CAMPS ON THE KETTLE VALLEY LINE, 1910 TO 1914 by Carson Toews B.A., The University of British Columbia, 2019 A THESIS SUBMITTED IN PARTIAL FULFILLMENT OF THE REQUIREMENTS FOR THE DEGREE OF MASTER OF ARTS in THE COLLEGE OF GRADUATE STUDIES (Interdisciplinary Studies) THE UNIVERSITY OF BRITISH COLUMBIA (Okanagan) November 2019 © Carson Daniel Toews, 2019 The following individuals certify that they have read, and recommend to the College of Graduate Studies for acceptance, a thesis/dissertation entitled: The Life of a Navvy: A Study of the Relationship Between Ethnicity and Status Within Railway Work Camps on the Kettle Valley Line, 1910 to 1914 submitted by Carson Toews in partial fulfillment of the requirements of the degree of Master of Arts . Christine Schreyer Supervisor Robin Dods Supervisory Committee Member Victoria Castillo Supervisory Committee Member Nancy Holmes University Examiner ii Abstract The majority of historical and archaeological studies of western Canadian railways have focused on nineteenth century Chinese immigrant workers. Alternatively, I examine the ethnicity and status of early twentieth century European immigrant railway workers in British Columbia by studying the men who constructed the Myra Canyon section of the Kettle Valley Railway (KVR) from 1910 to 1914. The primary function of the KVR was to supply a trade link for mining operations in the Interior region of BC. An important local historical landmark, the 12 km long Myra Canyon section of the KVR is located within the Myra-Bellevue Provincial Park and is comprised of two rock tunnels and 18 wooden trestles through the rugged terrain south- east of the City of Kelowna.