National Advisory Commitiee for Aeronautics
Total Page:16
File Type:pdf, Size:1020Kb
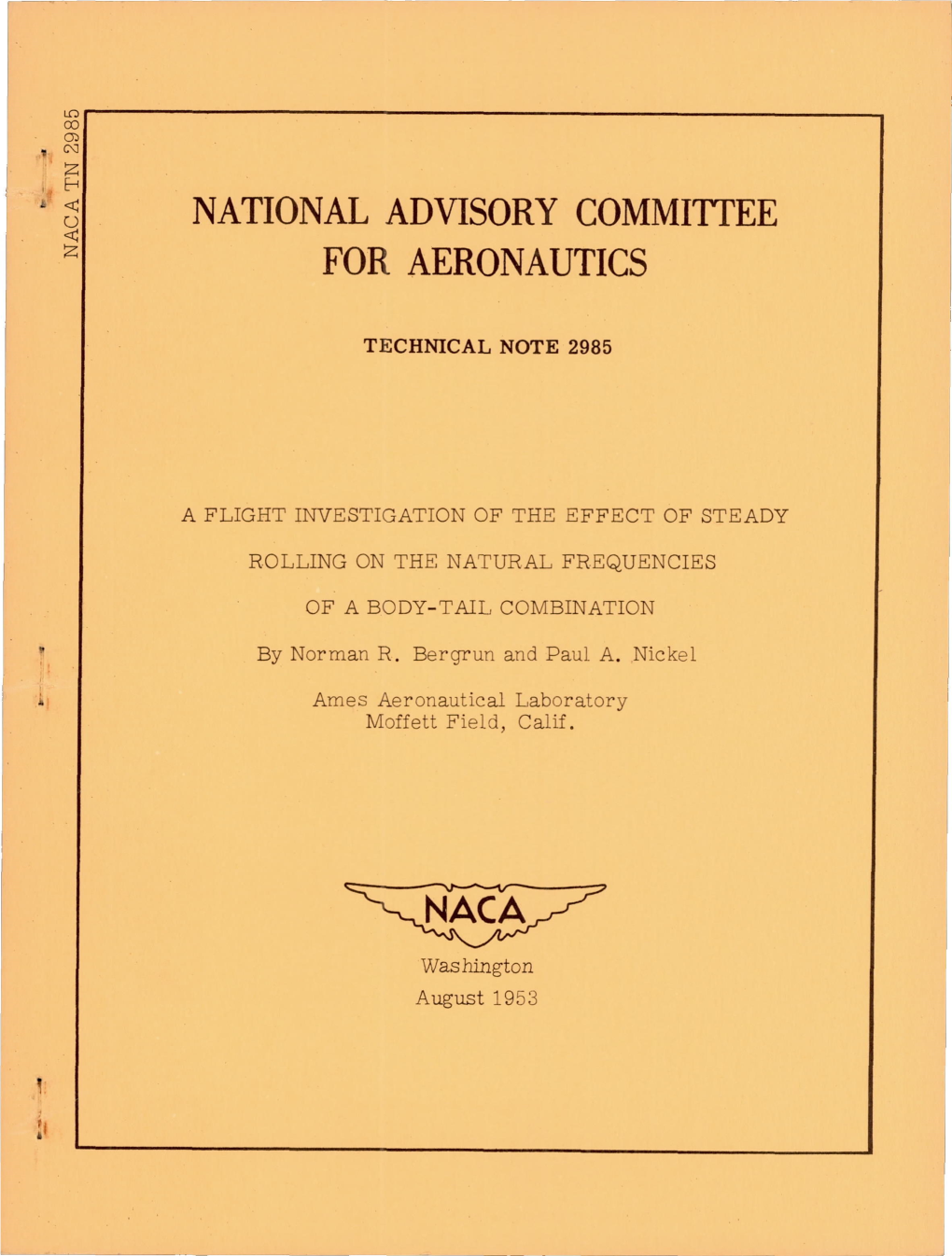
Load more
Recommended publications
-
Linear and Angular Velocity Examples
Linear and Angular Velocity Examples Example 1 Determine the angular displacement in radians of 6.5 revolutions. Round to the nearest tenth. Each revolution equals 2 radians. For 6.5 revolutions, the number of radians is 6.5 2 or 13 . 13 radians equals about 40.8 radians. Example 2 Determine the angular velocity if 4.8 revolutions are completed in 4 seconds. Round to the nearest tenth. The angular displacement is 4.8 2 or 9.6 radians. = t 9.6 = = 9.6 , t = 4 4 7.539822369 Use a calculator. The angular velocity is about 7.5 radians per second. Example 3 AMUSEMENT PARK Jack climbs on a horse that is 12 feet from the center of a merry-go-round. 1 The merry-go-round makes 3 rotations per minute. Determine Jack’s angular velocity in radians 4 per second. Round to the nearest hundredth. 1 The merry-go-round makes 3 or 3.25 revolutions per minute. Convert revolutions per minute to radians 4 per second. 3.25 revolutions 1 minute 2 radians 0.3403392041 radian per second 1 minute 60 seconds 1 revolution Jack has an angular velocity of about 0.34 radian per second. Example 4 Determine the linear velocity of a point rotating at an angular velocity of 12 radians per second at a distance of 8 centimeters from the center of the rotating object. Round to the nearest tenth. v = r v = (8)(12 ) r = 8, = 12 v 301.5928947 Use a calculator. The linear velocity is about 301.6 centimeters per second. -
Guide for the Use of the International System of Units (SI)
Guide for the Use of the International System of Units (SI) m kg s cd SI mol K A NIST Special Publication 811 2008 Edition Ambler Thompson and Barry N. Taylor NIST Special Publication 811 2008 Edition Guide for the Use of the International System of Units (SI) Ambler Thompson Technology Services and Barry N. Taylor Physics Laboratory National Institute of Standards and Technology Gaithersburg, MD 20899 (Supersedes NIST Special Publication 811, 1995 Edition, April 1995) March 2008 U.S. Department of Commerce Carlos M. Gutierrez, Secretary National Institute of Standards and Technology James M. Turner, Acting Director National Institute of Standards and Technology Special Publication 811, 2008 Edition (Supersedes NIST Special Publication 811, April 1995 Edition) Natl. Inst. Stand. Technol. Spec. Publ. 811, 2008 Ed., 85 pages (March 2008; 2nd printing November 2008) CODEN: NSPUE3 Note on 2nd printing: This 2nd printing dated November 2008 of NIST SP811 corrects a number of minor typographical errors present in the 1st printing dated March 2008. Guide for the Use of the International System of Units (SI) Preface The International System of Units, universally abbreviated SI (from the French Le Système International d’Unités), is the modern metric system of measurement. Long the dominant measurement system used in science, the SI is becoming the dominant measurement system used in international commerce. The Omnibus Trade and Competitiveness Act of August 1988 [Public Law (PL) 100-418] changed the name of the National Bureau of Standards (NBS) to the National Institute of Standards and Technology (NIST) and gave to NIST the added task of helping U.S. -
TECHNICAL Nbte D-626
NASA TN D-626 LIBRARY COPY NOV 16 1960 SPACE FLIGHT LANGLEY FIELD, VIRGINIA t TECHNICAL NbTE D-626 SATELLITE ATTDUDE CONTROL USING A COMBINATION OF INERTIA WHEELS AND A BAR MAGNET By James J. Adams and Roy F. Brissenden Langley Research Center Langley Field, Va. NATIONAL AERONAUTICS AND SPACE ADMINISTRATION WASHINGTON November 1960 . NATIONAL AERONAUTICS AND SPACE ADMINISTRATION TECHNICAL NOTE D-626 SATELLITE ATTITUDE CONTROL USING A COMBINATION OF INERTIA WHEELS AND A BAR MAGNET By James J. Adams and Roy F. Brissenden SUMMARY The use of inertia wheels to control the attitude of a satellite has currently aroused much interest. The stability of such a system has been studied in this investigation. A single-degree-of-freedom analysis indicates that a response with suitable dynamic characteristics and pre- cise control can be achieved by commanding the angular velocity of the inertia wheel with an error signal that is the sum of the attitude error, the attitude rate, and the integral of the attitude error. A digital computer was used to study the three-degree-of-freedom response to step displacements, and the results indicate that the cross-coupling effects of inertia coupling and precession coupling had no effect on system sta- bility. A study was also made of the use of a bar magnet to supplement the inertia wheels by providing a means of removing any momentum intro- duced into the system by disturbances such as aerodynamic torques. A study of a case with large aerodynamic torques, with a typical orbit, indicated that the magnet was a suitable device for supplying the essen- tial trimming force. -
The International System of Units (SI) - Conversion Factors For
NIST Special Publication 1038 The International System of Units (SI) – Conversion Factors for General Use Kenneth Butcher Linda Crown Elizabeth J. Gentry Weights and Measures Division Technology Services NIST Special Publication 1038 The International System of Units (SI) - Conversion Factors for General Use Editors: Kenneth S. Butcher Linda D. Crown Elizabeth J. Gentry Weights and Measures Division Carol Hockert, Chief Weights and Measures Division Technology Services National Institute of Standards and Technology May 2006 U.S. Department of Commerce Carlo M. Gutierrez, Secretary Technology Administration Robert Cresanti, Under Secretary of Commerce for Technology National Institute of Standards and Technology William Jeffrey, Director Certain commercial entities, equipment, or materials may be identified in this document in order to describe an experimental procedure or concept adequately. Such identification is not intended to imply recommendation or endorsement by the National Institute of Standards and Technology, nor is it intended to imply that the entities, materials, or equipment are necessarily the best available for the purpose. National Institute of Standards and Technology Special Publications 1038 Natl. Inst. Stand. Technol. Spec. Pub. 1038, 24 pages (May 2006) Available through NIST Weights and Measures Division STOP 2600 Gaithersburg, MD 20899-2600 Phone: (301) 975-4004 — Fax: (301) 926-0647 Internet: www.nist.gov/owm or www.nist.gov/metric TABLE OF CONTENTS FOREWORD.................................................................................................................................................................v -
Some Considerations on Quantities of Dimension One in Oscillatory Motions
Some considerations on quantities of dimension one in oscillatory motions Leonardo Gariboldi Dipartimento di Fisica, Università degli Studi di Milano, Via Celoria 16, 20133, Milano, Italy. E-mail: [email protected] (Received 13 February 2012, accepted 27 May 2012) Abstract The habit of not writing a multiplicative factor of dimension one leads to equations, which, though dimensionally correct, can pose some problems to most students as for the analysis of the units of measurement of the written physical quantities. The proposed analysis of the derivation of the equations for a simple oscillator and a forced oscillator with damping shows how to handle with the units of measurement. Similar considerations can be done for the formula of error propagation for oscillating functions. Keywords: Units of measurement, Radian, Oscillatory motion. Resumen El hábito de no escribir un factor multiplicativo de una dimensión conduce a las ecuaciones, las cuales, aunque de dimensiones correctas, pueden plantear algunos problemas a la mayoría de los estudiantes como para el análisis de las unidades de medida de las cantidades físicas escritas. El análisis propuesto de la derivación de las ecuaciones para un oscilador simple y un oscilador forzado con amortiguamiento muestra cómo controlar a las unidades de medida. Se pueden hacer consideraciones similares para la fórmula de propagación de errores para funciones oscilantes. Palabras clave: Unidades de medición, Radián, Movimiento oscilatorio. PACS: 01.40.Fk, 01.40.gb, O6.20.Dk ISSN 1870-9095 I. INTRODUCTION II. A PROBLEM WITH THE UNIT OF MEASUREMENT OF THE ANGULAR The study of oscillatory motions in the most common FREQUENCY physics textbooks used in undergraduate courses (see, e.g., [1, 2, 3, 4, 5]) is based on definitions and laws described in The frequency ν of an oscillatory motion is defined as the previous chapters (e.g.: Cinematic, Newton’s laws) and on number of oscillation cycles per time. -
Technical Standards Board Standard
TECHNICAL REV. STANDARDS TSB 003 MAY1999 BOARD Issued 1965-06 400 Commonwealth Drive, Warrendale, PA 15096-0001 STANDARD Revised 1999-05 Superseding TSB 003 JUN92 Submitted for recognition as an American National Standard Rules for SAE Use of SI (Metric) Units Foreword—SI denotes The International System of Units (Le Système International d’Unités). SI was established in 1960, under the Treaty of the Meter, by Resolutions and Recommendations of the General Conference on Weights and Measures (Conférence Générale des Poids et Mesures, CGPM) and the International Committee for Weights and Measures (Comité International des Poids et Mesures, CIPM) on The International System of Units. The abbreviation "SI" is used in all languages. In 1969, the SAE Board of Directors issued a directive that "SAE will include SI units in SAE Standards and other technical reports." During the ensuing several decades, SAE metric policy evolved and implementation progressed. The SAE’s current metric policy is, "Operating Boards shall not use any weights and measures system other than metric (SI), except when conversion is not practical, or where a conflicting world industry practice exists." Principal driving forces for SAE metrication were: worldwide movement to metric units; enactment of United States Federal metric legislation and the resultant national metrication activity; the international trend in industry and business throughout the world, and the growing international scope of SAE. Currently, the widespread, strong support for international standards harmonization is another key motivating factor in the global metrication movement. TSB 003 (formerly SAE J916) has been updated periodically, to reflect SAE metric policy evolution—as well as developments in the specific, formal content of SI; and in the correct, consistent usage and application of SI...which sometimes is referred to as "the modern version of the metric measurement system." The content of TSB 003 is consistent with international and U.S. -