Aurora-A Phosphorylates Splicing Factors and Regulates Alternative Splicing
Total Page:16
File Type:pdf, Size:1020Kb
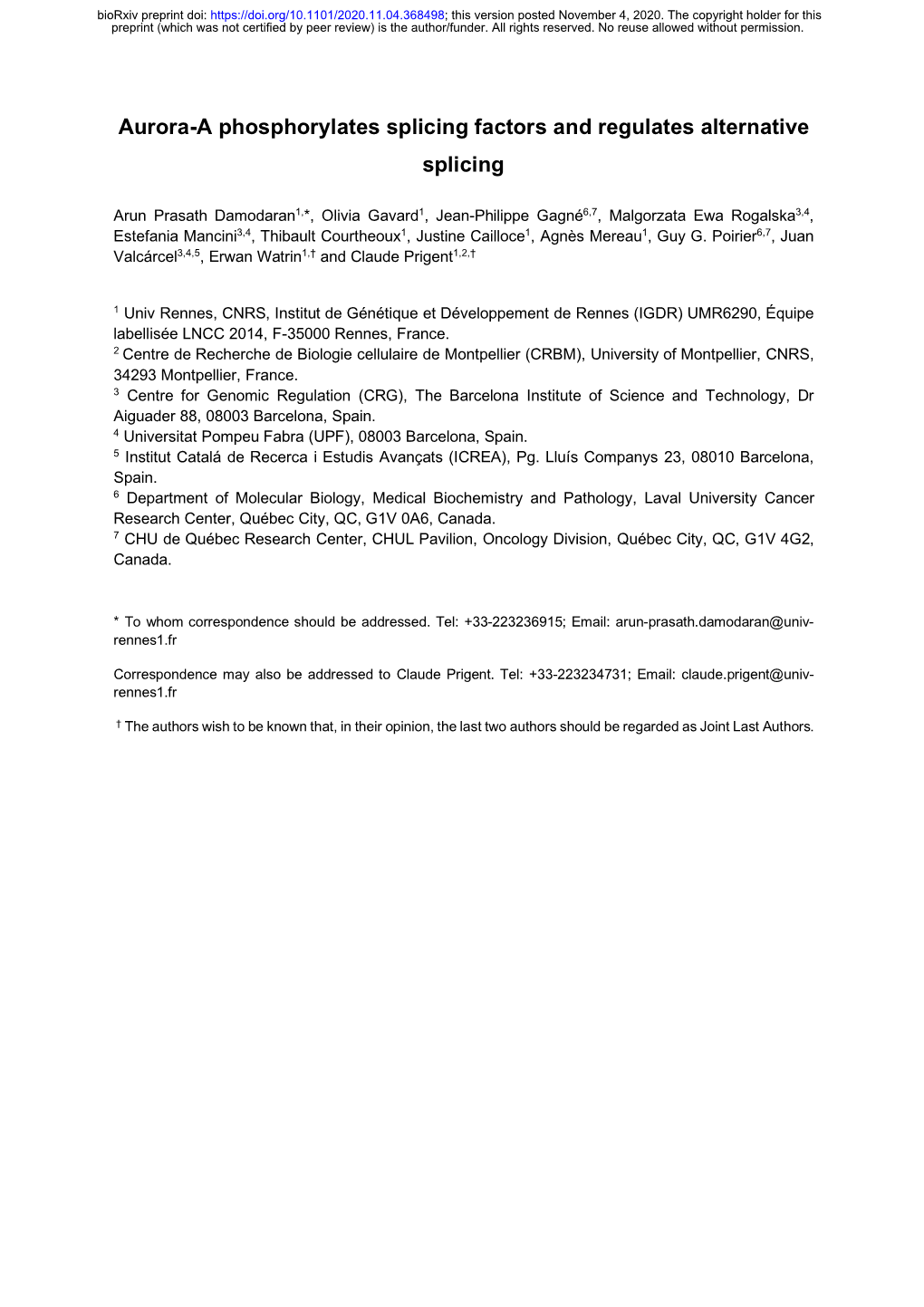
Load more
Recommended publications
-
Supplemental Material Table of Contents
Supplemental material Table of Contents Detailed Materials and Methods ......................................................................................................... 2 Perioperative period ........................................................................................................................... 2 Ethical aspects ................................................................................................................................... 4 Evaluation of heart failure ................................................................................................................. 4 Sample preparation for ANP mRNA expression .................................................................................. 5 Sample preparation for validative qRT-PCR (Postn, Myh7, Gpx3, Tgm2) ............................................ 6 Tissue fibrosis .................................................................................................................................... 7 Ventricular remodeling and histological tissue preservation ................................................................ 8 Evaluation of the histological preservation of cardiac tissue ................................................................ 9 Sample preparation and quantitative label-free proteomics analyses .................................................. 10 Statistical methods ........................................................................................................................... 12 References ........................................................................................................................................ -
Datasheet: VPA00331KT Product Details
Datasheet: VPA00331KT Description: PRPF19 ANTIBODY WITH CONTROL LYSATE Specificity: PRPF19 Format: Purified Product Type: PrecisionAb™ Polyclonal Isotype: Polyclonal IgG Quantity: 2 Westerns Product Details Applications This product has been reported to work in the following applications. This information is derived from testing within our laboratories, peer-reviewed publications or personal communications from the originators. Please refer to references indicated for further information. For general protocol recommendations, please visit www.bio-rad-antibodies.com/protocols. Yes No Not Determined Suggested Dilution Western Blotting 1/1000 PrecisionAb antibodies have been extensively validated for the western blot application. The antibody has been validated at the suggested dilution. Where this product has not been tested for use in a particular technique this does not necessarily exclude its use in such procedures. Further optimization may be required dependant on sample type. Target Species Human Species Cross Reacts with: Mouse, Rat Reactivity N.B. Antibody reactivity and working conditions may vary between species. Product Form Purified IgG - liquid Preparation 20μl Rabbit polyclonal antibody purified by affinity chromatography Buffer Solution Phosphate buffered saline Preservative 0.09% Sodium Azide (NaN ) Stabilisers 3 Immunogen KLH-conjugated synthetic peptide corresponding to aa 4-33 of human PRPF19 External Database Links UniProt: Q9UMS4 Related reagents Entrez Gene: 27339 PRPF19 Related reagents Synonyms NMP200, PRP19, SNEV Page 1 of 3 Specificity Rabbit anti Human PRPF19 antibody recognizes PRPF19, also known as PRP19/PSO4 homolog, PRP19/PSO4 pre-mRNA processing factor 19 homolog, nuclear matrix protein 200, nuclear matrix protein NMP200 related to splicing factor PRP19, psoralen 4 and senescence evasion factor. The PRPF19 gene is the human homolog of yeast Pso4, a gene essential for cell survival and DNA repair (Beck et al. -
A Computational Approach for Defining a Signature of Β-Cell Golgi Stress in Diabetes Mellitus
Page 1 of 781 Diabetes A Computational Approach for Defining a Signature of β-Cell Golgi Stress in Diabetes Mellitus Robert N. Bone1,6,7, Olufunmilola Oyebamiji2, Sayali Talware2, Sharmila Selvaraj2, Preethi Krishnan3,6, Farooq Syed1,6,7, Huanmei Wu2, Carmella Evans-Molina 1,3,4,5,6,7,8* Departments of 1Pediatrics, 3Medicine, 4Anatomy, Cell Biology & Physiology, 5Biochemistry & Molecular Biology, the 6Center for Diabetes & Metabolic Diseases, and the 7Herman B. Wells Center for Pediatric Research, Indiana University School of Medicine, Indianapolis, IN 46202; 2Department of BioHealth Informatics, Indiana University-Purdue University Indianapolis, Indianapolis, IN, 46202; 8Roudebush VA Medical Center, Indianapolis, IN 46202. *Corresponding Author(s): Carmella Evans-Molina, MD, PhD ([email protected]) Indiana University School of Medicine, 635 Barnhill Drive, MS 2031A, Indianapolis, IN 46202, Telephone: (317) 274-4145, Fax (317) 274-4107 Running Title: Golgi Stress Response in Diabetes Word Count: 4358 Number of Figures: 6 Keywords: Golgi apparatus stress, Islets, β cell, Type 1 diabetes, Type 2 diabetes 1 Diabetes Publish Ahead of Print, published online August 20, 2020 Diabetes Page 2 of 781 ABSTRACT The Golgi apparatus (GA) is an important site of insulin processing and granule maturation, but whether GA organelle dysfunction and GA stress are present in the diabetic β-cell has not been tested. We utilized an informatics-based approach to develop a transcriptional signature of β-cell GA stress using existing RNA sequencing and microarray datasets generated using human islets from donors with diabetes and islets where type 1(T1D) and type 2 diabetes (T2D) had been modeled ex vivo. To narrow our results to GA-specific genes, we applied a filter set of 1,030 genes accepted as GA associated. -
Identification and Characterization of Genes Essential for Human Brain Development
Identification and Characterization of Genes Essential for Human Brain Development The Harvard community has made this article openly available. Please share how this access benefits you. Your story matters Citation Ganesh, Vijay S. 2012. Identification and Characterization of Genes Essential for Human Brain Development. Doctoral dissertation, Harvard University. Citable link http://nrs.harvard.edu/urn-3:HUL.InstRepos:9773743 Terms of Use This article was downloaded from Harvard University’s DASH repository, and is made available under the terms and conditions applicable to Other Posted Material, as set forth at http:// nrs.harvard.edu/urn-3:HUL.InstRepos:dash.current.terms-of- use#LAA Copyright © 2012 by Vijay S. Ganesh All rights reserved. Dissertation Advisor: Dr. Christopher A. Walsh Author: Vijay S. Ganesh Identification and Characterization of Genes Essential for Human Brain Development Abstract The human brain is a network of ninety billion neurons that allows for many of the behavioral adaptations considered unique to our species. One-fifth of these neurons are layered in an epithelial sheet known as the cerebral cortex, which is exquisitely folded into convolutions called gyri. Defects in neuronal number clinically present with microcephaly (Greek for “small head”), and in inherited cases these defects can be linked to mutations that identify genes essential for neural progenitor proliferation. Most microcephaly genes are characterized to play a role in the centrosome, however rarer presentations of microcephaly have identified different mechanisms. Charged multivesicular body protein/Chromatin modifying protein 1A (CHMP1A) is a member of the ESCRT-III endosomal sorting complex, but is also suggested to localize to the nuclear matrix and regulate chromatin. -
Molecular Genetics of Microcephaly Primary Hereditary: an Overview
brain sciences Review Molecular Genetics of Microcephaly Primary Hereditary: An Overview Nikistratos Siskos † , Electra Stylianopoulou †, Georgios Skavdis and Maria E. Grigoriou * Department of Molecular Biology & Genetics, Democritus University of Thrace, 68100 Alexandroupolis, Greece; [email protected] (N.S.); [email protected] (E.S.); [email protected] (G.S.) * Correspondence: [email protected] † Equal contribution. Abstract: MicroCephaly Primary Hereditary (MCPH) is a rare congenital neurodevelopmental disorder characterized by a significant reduction of the occipitofrontal head circumference and mild to moderate mental disability. Patients have small brains, though with overall normal architecture; therefore, studying MCPH can reveal not only the pathological mechanisms leading to this condition, but also the mechanisms operating during normal development. MCPH is genetically heterogeneous, with 27 genes listed so far in the Online Mendelian Inheritance in Man (OMIM) database. In this review, we discuss the role of MCPH proteins and delineate the molecular mechanisms and common pathways in which they participate. Keywords: microcephaly; MCPH; MCPH1–MCPH27; molecular genetics; cell cycle 1. Introduction Citation: Siskos, N.; Stylianopoulou, Microcephaly, from the Greek word µικρoκεϕαλi´α (mikrokephalia), meaning small E.; Skavdis, G.; Grigoriou, M.E. head, is a term used to describe a cranium with reduction of the occipitofrontal head circum- Molecular Genetics of Microcephaly ference equal, or more that teo standard deviations -
Nuclear PTEN Safeguards Pre-Mrna Splicing to Link Golgi Apparatus for Its Tumor Suppressive Role
ARTICLE DOI: 10.1038/s41467-018-04760-1 OPEN Nuclear PTEN safeguards pre-mRNA splicing to link Golgi apparatus for its tumor suppressive role Shao-Ming Shen1, Yan Ji2, Cheng Zhang1, Shuang-Shu Dong2, Shuo Yang1, Zhong Xiong1, Meng-Kai Ge1, Yun Yu1, Li Xia1, Meng Guo1, Jin-Ke Cheng3, Jun-Ling Liu1,3, Jian-Xiu Yu1,3 & Guo-Qiang Chen1 Dysregulation of pre-mRNA alternative splicing (AS) is closely associated with cancers. However, the relationships between the AS and classic oncogenes/tumor suppressors are 1234567890():,; largely unknown. Here we show that the deletion of tumor suppressor PTEN alters pre-mRNA splicing in a phosphatase-independent manner, and identify 262 PTEN-regulated AS events in 293T cells by RNA sequencing, which are associated with significant worse outcome of cancer patients. Based on these findings, we report that nuclear PTEN interacts with the splicing machinery, spliceosome, to regulate its assembly and pre-mRNA splicing. We also identify a new exon 2b in GOLGA2 transcript and the exon exclusion contributes to PTEN knockdown-induced tumorigenesis by promoting dramatic Golgi extension and secretion, and PTEN depletion significantly sensitizes cancer cells to secretion inhibitors brefeldin A and golgicide A. Our results suggest that Golgi secretion inhibitors alone or in combination with PI3K/Akt kinase inhibitors may be therapeutically useful for PTEN-deficient cancers. 1 Department of Pathophysiology, Key Laboratory of Cell Differentiation and Apoptosis of Chinese Ministry of Education, Shanghai Jiao Tong University School of Medicine (SJTU-SM), Shanghai 200025, China. 2 Institute of Health Sciences, Shanghai Institutes for Biological Sciences of Chinese Academy of Sciences and SJTU-SM, Shanghai 200025, China. -
Ncomms4885.Pdf
ARTICLE Received 4 Feb 2014 | Accepted 14 Apr 2014 | Published 30 May 2014 DOI: 10.1038/ncomms4885 Microcephaly disease gene Wdr62 regulates mitotic progression of embryonic neural stem cells and brain size Jian-Fu Chen1,2, Ying Zhang1, Jonathan Wilde1, Kirk C. Hansen3, Fan Lai4 & Lee Niswander1 Human genetic studies have established a link between a class of centrosome proteins and microcephaly. Current studies of microcephaly focus on defective centrosome/spindle orientation. Mutations in WDR62 are associated with microcephaly and other cortical abnormalities in humans. Here we create a mouse model of Wdr62 deficiency and find that the mice exhibit reduced brain size due to decreased neural progenitor cells (NPCs). Wdr62 depleted cells show spindle instability, spindle assembly checkpoint (SAC) activation, mitotic arrest and cell death. Mechanistically, Wdr62 associates and genetically interacts with Aurora A to regulate spindle formation, mitotic progression and brain size. Our results suggest that Wdr62 interacts with Aurora A to control mitotic progression, and loss of these interactions leads to mitotic delay and cell death of NPCs, which could be a potential cause of human microcephaly. 1 Howard Hughes Medical Institute, Department of Pediatrics, University of Colorado Anschutz Medical Campus, Children’s Hospital Colorado, Aurora, Colorado 80045, USA. 2 Department of Genetics, Department of Biochemistry & Molecular Biology, University of Georgia, Athens, Georgia 30602, USA. 3 Biochemistry & Molecular Genetics, University of Colorado Denver, Aurora, Colorado 80045, USA. 4 The Wistar Institute, 3601 Spruce Street, Philadelphia, Pennsylvania 19104, USA. Correspondence and requests for materials should be addressed to J-F.C. (email: [email protected]) or to L.N. -
Human Prefoldin Modulates Co-Transcriptional Pre-Mrna Splicing
bioRxiv preprint doi: https://doi.org/10.1101/2020.06.14.150466; this version posted July 22, 2020. The copyright holder for this preprint (which was not certified by peer review) is the author/funder. All rights reserved. No reuse allowed without permission. BIOLOGICAL SCIENCES: Biochemistry Human prefoldin modulates co-transcriptional pre-mRNA splicing Payán-Bravo L 1,2, Peñate X 1,2 *, Cases I 3, Pareja-Sánchez Y 1, Fontalva S 1,2, Odriozola Y 1,2, Lara E 1, Jimeno-González S 2,5, Suñé C 4, Reyes JC 5, Chávez S 1,2. 1 Instituto de Biomedicina de Sevilla, Universidad de Sevilla-CSIC-Hospital Universitario V. del Rocío, Seville, Spain. 2 Departamento de Genética, Facultad de Biología, Universidad de Sevilla, Seville, Spain. 3 Centro Andaluz de Biología del Desarrollo, CSIC-Universidad Pablo de Olavide, Seville, Spain. 4 Department of Molecular Biology, Institute of Parasitology and Biomedicine "López Neyra" IPBLN-CSIC, PTS, Granada, Spain. 5 Andalusian Center of Molecular Biology and Regenerative Medicine-CABIMER, Junta de Andalucia-University of Pablo de Olavide-University of Seville-CSIC, Seville, Spain. Correspondence: Sebastián Chávez, IBiS, campus HUVR, Avda. Manuel Siurot s/n, Sevilla, 41013, Spain. Phone: +34-955923127: e-mail: [email protected]. * Co- corresponding; [email protected]. bioRxiv preprint doi: https://doi.org/10.1101/2020.06.14.150466; this version posted July 22, 2020. The copyright holder for this preprint (which was not certified by peer review) is the author/funder. All rights reserved. No reuse allowed without permission. Abstract Prefoldin is a heterohexameric complex conserved from archaea to humans that plays a cochaperone role during the cotranslational folding of actin and tubulin monomers. -
(JNK)-Binding Protein WDR62 Is Recruited to Stress Granules And
Molecular Biology of the Cell Vol. 21, 117–130, January 1, 2010 A Novel c-Jun N-terminal Kinase (JNK)-binding Protein WDR62 Is Recruited to Stress Granules and Mediates a Nonclassical JNK Activation Tanya Wasserman,*† Ksenya Katsenelson,*† Sharon Daniliuc,*† Tal Hasin,* Mordechay Choder,‡ and Ami Aronheim* Departments of *Molecular Genetics and ‡Microbiology, The Rappaport Family Institute for Research in the Medical Sciences, Technion-Israel Institute of Technology, Haifa 31096, Israel Submitted June 22, 2009; Revised October 27, 2009; Accepted October 29, 2009 Monitoring Editor: Jonathan Chernoff The c-Jun N-terminal kinase (JNK) is part of a mitogen-activated protein kinase (MAPK) signaling cascade. Scaffold proteins simultaneously associate with various components of the MAPK signaling pathway and play a role in signal transmission and regulation. Here we describe the identification of a novel scaffold JNK-binding protein, WDR62, with no sequence homology to any of the known scaffold proteins. WDR62 is a ubiquitously expressed heat-sensitive 175-kDa protein that specifically associates with JNK but not with ERK and p38. Association between WDR62 and JNKs occurs in the absence and after either transient or persistent stimuli. WDR62 potentiates JNK kinase activity; however it inhibits AP-1 transcription through recruitment of JNK to a nonnuclear compartment. HEK-293T cells transfected with WDR62 display cytoplasmic granular localization. Overexpression of stress granule (SG) resident proteins results in the recruit- ment of endogenous WDR62 and activated JNK to SG. In addition, cell treatment with arsenite results in recruitment of WDR62 to SG and activated JNK to processing bodies (PB). JNK inhibition results in reduced number and size of SG and reduced size of PB. -
Cenpj Regulates Cilia Disassembly and Neurogenesis in the Developing Mouse Cortex
This Accepted Manuscript has not been copyedited and formatted. The final version may differ from this version. A link to any extended data will be provided when the final version is posted online. Research Articles: Development/Plasticity/Repair Cenpj regulates cilia disassembly and neurogenesis in the developing mouse cortex Wenyu Ding1,2, Qian Wu1,2, Le Sun1,2, Na Clara Pan1,2 and Xiaoqun Wang1,2,2 1State Key Laboratory of Brain and Cognitive Science, CAS Center for Excellence in Brain Science and Intelligence Technology (Shanghai), Institute of Biophysics, Chinese Academy of Sciences, Beijing, 100101, China 2University of Chinese Academy of Sciences, Beijing 100049, China 3Beijing Institute for Brain Disorders, Beijing 100069, China https://doi.org/10.1523/JNEUROSCI.1849-18.2018 Received: 20 July 2018 Revised: 19 December 2018 Accepted: 24 December 2018 Published: 9 January 2019 Author contributions: W.D., Q.W., and X.W. designed research; W.D., Q.W., L.S., N.C.P., and X.W. performed research; W.D., Q.W., L.S., N.C.P., and X.W. analyzed data; W.D., Q.W., L.S., N.C.P., and X.W. edited the paper; W.D., Q.W., and X.W. wrote the paper; Q.W. and X.W. wrote the first draft of the paper; X.W. contributed unpublished reagents/analytic tools. Conflict of Interest: The authors declare no competing financial interests. We gratefully acknowledge Dr. Bradley Yoder (University of Alabama at Birmingham) kindly shared and transferred the mouse strain to us. We thank Dr. Tian Xue (University of Science and Technology of China) for sharing ARPE19 cell line with us. -
Mrna Editing, Processing and Quality Control in Caenorhabditis Elegans
| WORMBOOK mRNA Editing, Processing and Quality Control in Caenorhabditis elegans Joshua A. Arribere,*,1 Hidehito Kuroyanagi,†,1 and Heather A. Hundley‡,1 *Department of MCD Biology, UC Santa Cruz, California 95064, †Laboratory of Gene Expression, Medical Research Institute, Tokyo Medical and Dental University, Tokyo 113-8510, Japan, and ‡Medical Sciences Program, Indiana University School of Medicine-Bloomington, Indiana 47405 ABSTRACT While DNA serves as the blueprint of life, the distinct functions of each cell are determined by the dynamic expression of genes from the static genome. The amount and specific sequences of RNAs expressed in a given cell involves a number of regulated processes including RNA synthesis (transcription), processing, splicing, modification, polyadenylation, stability, translation, and degradation. As errors during mRNA production can create gene products that are deleterious to the organism, quality control mechanisms exist to survey and remove errors in mRNA expression and processing. Here, we will provide an overview of mRNA processing and quality control mechanisms that occur in Caenorhabditis elegans, with a focus on those that occur on protein-coding genes after transcription initiation. In addition, we will describe the genetic and technical approaches that have allowed studies in C. elegans to reveal important mechanistic insight into these processes. KEYWORDS Caenorhabditis elegans; splicing; RNA editing; RNA modification; polyadenylation; quality control; WormBook TABLE OF CONTENTS Abstract 531 RNA Editing and Modification 533 Adenosine-to-inosine RNA editing 533 The C. elegans A-to-I editing machinery 534 RNA editing in space and time 535 ADARs regulate the levels and fates of endogenous dsRNA 537 Are other modifications present in C. -
Functional Analysis of the Nuclear Basket and Protein Tpr
Haruki Iino (Autor) Functional analysis of the nuclear basket and protein Tpr https://cuvillier.de/de/shop/publications/7587 Copyright: Cuvillier Verlag, Inhaberin Annette Jentzsch-Cuvillier, Nonnenstieg 8, 37075 Göttingen, Germany Telefon: +49 (0)551 54724-0, E-Mail: [email protected], Website: https://cuvillier.de 2. Abstract A large coiled-coil protein, Tpr (Translocated promoter region) is located at the nuclear side of the nuclear pore complex (NPC), and plays an important role in the architecture of the nuclear basket (NB). Although its contribution to the NB structure has been characterized, little is known about the function of Tpr. In this work, we investigated whether and how Tpr contributes to the surveillance of mRNA export, especially, to the quality control (QC) of certain un-spliced mRNAs. To answer these questions, we established a series of different reporter cell lines, allowing us to monitor the occurrence of un-spliced and spliced reporter transcripts. In the analysis of most of these reporter cell lines, the depletion of Tpr did not cause any obvious leakage of un-spliced reporter mRNAs. However, when the coding sequence of the HIV (Human immunodeficiency virus) Gag protein was used as a readout, the cytoplasmic levels of the un-spliced HIV-gag mRNAs were highly enhanced in the absence of Tpr. Such phenotype was only observed when a viral RNA export enhancer element, the CTE (the constitutive transport element), that recruits the general mRNA export receptor, NXF1/TAP, was part of the HIV-gag reporter gene. Intriguingly, a slight reduction in the cellular Tpr levels already caused the breakdown of a retention mechanism that normally keeps these HIV-gag transcripts in the nucleus.