Simulations of the Transformation Stage of the Extratropical Transition of Tropical Cyclones
Total Page:16
File Type:pdf, Size:1020Kb
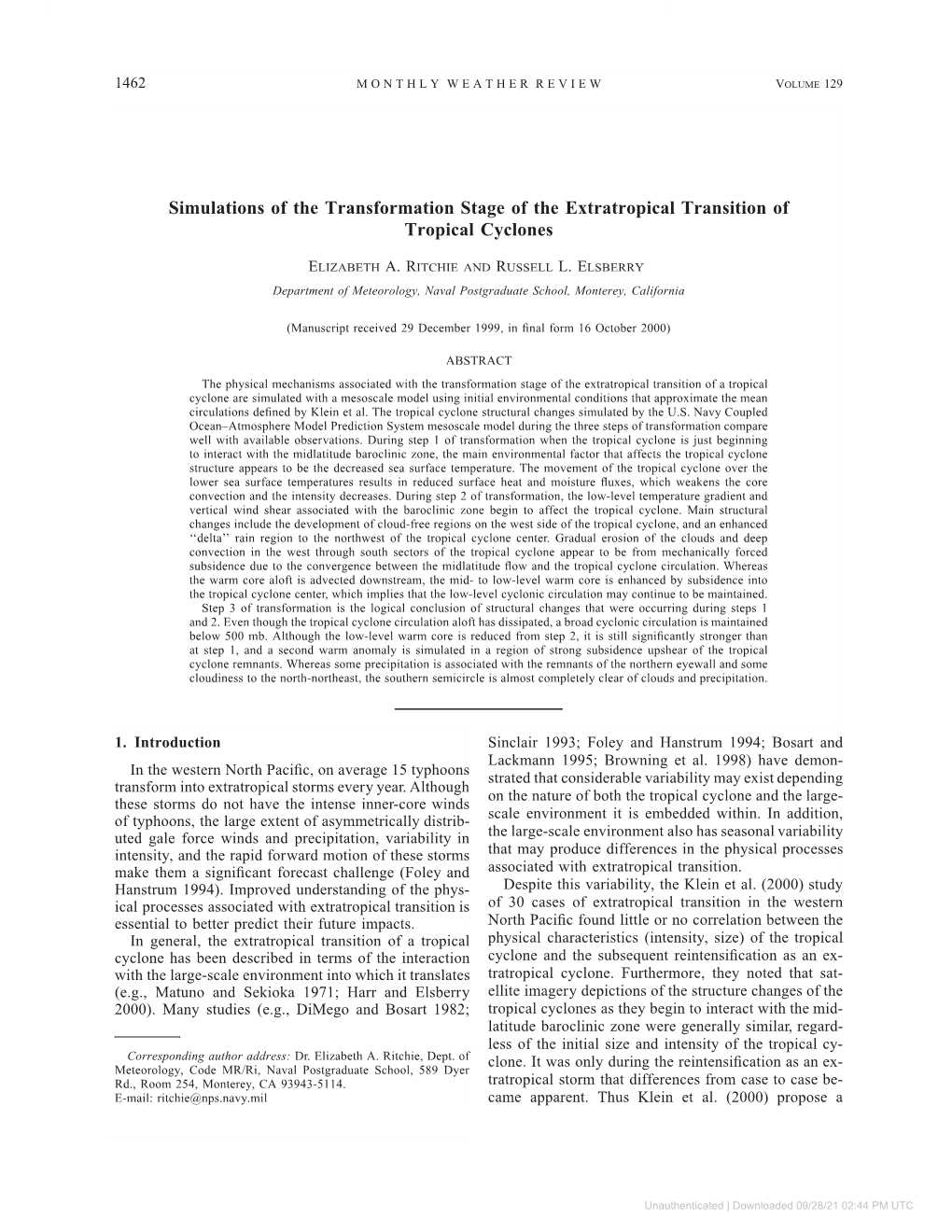
Load more
Recommended publications
-
Typhoon Neoguri Disaster Risk Reduction Situation Report1 DRR Sitrep 2014‐001 ‐ Updated July 8, 2014, 10:00 CET
Typhoon Neoguri Disaster Risk Reduction Situation Report1 DRR sitrep 2014‐001 ‐ updated July 8, 2014, 10:00 CET Summary Report Ongoing typhoon situation The storm had lost strength early Tuesday July 8, going from the equivalent of a Category 5 hurricane to a Category 3 on the Saffir‐Simpson Hurricane Wind Scale, which means devastating damage is expected to occur, with major damage to well‐built framed homes, snapped or uprooted trees and power outages. It is approaching Okinawa, Japan, and is moving northwest towards South Korea and the Philippines, bringing strong winds, flooding rainfall and inundating storm surge. Typhoon Neoguri is a once‐in‐a‐decade storm and Japanese authorities have extended their highest storm alert to Okinawa's main island. The Global Assessment Report (GAR) 2013 ranked Japan as first among countries in the world for both annual and maximum potential losses due to cyclones. It is calculated that Japan loses on average up to $45.9 Billion due to cyclonic winds every year and that it can lose a probable maximum loss of $547 Billion.2 What are the most devastating cyclones to hit Okinawa in recent memory? There have been 12 damaging cyclones to hit Okinawa since 1945. Sustaining winds of 81.6 knots (151 kph), Typhoon “Winnie” caused damages of $5.8 million in August 1997. Typhoon "Bart", which hit Okinawa in October 1999 caused damages of $5.7 million. It sustained winds of 126 knots (233 kph). The most damaging cyclone to hit Japan was Super Typhoon Nida (reaching a peak intensity of 260 kph), which struck Japan in 2004 killing 287 affecting 329,556 people injuring 1,483, and causing damages amounting to $15 Billion. -
Intensification and Structure Change of Super Typhoon Flo As Related to the Large-Scale Environment
N PS ARCHIVE 1998.06 TITLEY, D. NAVAL POSTGRADUATE SCHOOL Monterey, California DISSERTATION INTENSIFICATION AND STRUCTURE CHANGE OF SUPER TYPHOON FLO AS RELATED TO THE LARGE-SCALE ENVIRONMENT by David W. Titley June 1998 Dissertation Supervisor: Russell L. Elsberry Thesis T5565 Approved for public release; distribution is unlimited. -EYKNC . •OOL DUDLEY KNOX LIBRARY NAVAL POSTGRADUATE SCHOOL MONTEREY, CA 93943-5101 REPORT DOCUMENTATION PAGE Form Approved OMB No 0704-01! Public reporting burden for this collection of information is estimated to average 1 hour per response, including the time for reviewing instruction, searching existing data sources, gathering and maintaining the data needed, and completing and reviewing the collection of information. Send comments regarding this burden estimate or any other aspect of this collection of information, including suggestions for reducing this burden, to Washington Headquarters Services, Directorate for Information Operations and Reports, 1215 Jefferson Davis Highway, Suite 1204, Arlington, VA 22202-4302, and to the Office of ManagemerJ and Budget, Paperwork Reduction Project (0704-0188) Washington DC 20503. 1. AGENCY USE ONLY (Leave blank) 2. REPORT DATE 3. REPORT TYPE AND DATES COVERED June 1998. Doctoral Dissertation 4. TITLE AND SUBTITLE Intensification and Structure Change of Super 5. FUNDING NUMBERS Typhoon Flo as Related to the Large-Scale Environment 6. AUTHOR(S) Titley, David W. PERFORMING ORGANIZATION NAME(S) AND ADDRESS(ES) PERFORMING Naval Postgraduate School ORGANIZATION Monterey CA 93943-5000 REPORT NUMBER 9. SPONSORING/MONITORING AGENCY NAME(S) AND ADDRESS(ES) 10. SPONSORING/MONITORING AGENCY REPORT NUMBER 11. SUPPLEMENTARY NOTES The views expressed in this thesis are those of the author and do not reflect the official policy or position of the Department of Defense or the U.S. -
1990) and Gene (1990
DECEMBER 1999 WU AND CHENG 3003 An Observational Study of Environmental In¯uences on the Intensity Changes of Typhoons Flo (1990) and Gene (1990) CHUN-CHIEH WUANDHSIU-JU CHENG Department of Atmospheric Sciences, National Taiwan University, Taipei, Taiwan (Manuscript received 27 July 1998, in ®nal form 22 December 1998) ABSTRACT The European Centre for Medium-Range Weather Forecasts Tropical Ocean±Global Atmosphere advanced analysis was used to study the mechanisms that affect the intensity of Typhoons Flo (1990) and Gene (1990). The out¯ow structure, eddy momentum ¯ux convergence, and the mean vertical wind shear were examined. The evolution of potential vorticity (PV) in the out¯ow layer showed low PV areas on top of both Typhoons Flo and Gene, and the low PV areas expanded as the typhoons intensi®ed. The out¯ow pattern of the two typhoons was in¯uenced by the upper-tropospheric environmental systems. The upper-level environmental fea- tures were shown to play a crucial role in the intensi®cation of the two typhoons. The tropical upper-tropospheric trough cell east of Flo provided the out¯ow channel for the typhoon. The enhanced out¯ow, the upper-level eddy ¯ux convergence (EFC), the low vertical wind shear, and the warm sea surface temperature provided all favorable conditions for the development of Flo. On the other hand, the intensi®cation of Gene was associated with its interaction with an upper-level midlatitude trough. The approach of the trough produced upper-level EFC of angular momentum outside 108 lat radius, and the EFC shifted inward with time. -
Large Intensity Changes in Tropical Cyclones: a Case Study of Supertyphoon Flo During TCM-90
3556 MONTHLY WEATHER REVIEW VOLUME 128 Large Intensity Changes in Tropical Cyclones: A Case Study of Supertyphoon Flo during TCM-90 DAVID W. T ITLEY AND RUSSELL L. ELSBERRY Department of Meteorology, Naval Postgraduate School, Monterey, California (Manuscript received 5 November 1998, in ®nal form 5 May 1999) ABSTRACT A unique dataset, recorded during the rapid intensi®cation and rapid decay of Typhoon Flo, is analyzed to isolate associated environmental conditions and key physical processes. This case occurred during the Tropical Cyclone Motion (TCM-90) ®eld experiment with enhanced observations, especially in the upper troposphere beyond about 300 km. These data have been analyzed with a four-dimensional data assimilation technique and a multiquadric interpolation technique. While both the ocean thermal structure and vertical wind shear are favorable, they do not explain the rapid intensi®cation or the rapid decay. A preconditioning phase is de®ned in which several interrelated factors combine to create favorable conditions: (i) a cyclonic wind burst occurs at 200 mb, (ii) vertical wind shear between 300 and 150 mb decreases 35%, (iii) the warm core is displaced upward, and (iv) 200-mb out¯ow becomes larger in the 400±1200-km radial band, while a layer of in¯ow develops below this out¯ow. These conditions appear to be forced by eddy ¯ux convergence (EFC) of angular momentum, which appears to act in a catalyst function as proposed by Pfeffer and colleagues, because the EFC then decreases to small values during the rapid intensi®cation stage. Similarly, the outer secondary circulation decreases during this stage, so that the rapid intensi®cation appears to be an internal (within 300 km) adjustment process that is perhaps triggered during the preconditioning phase. -
1990) and Gene (1990
DECEMBER 1999 WU AND CHENG 3003 An Observational Study of Environmental In¯uences on the Intensity Changes of Typhoons Flo (1990) and Gene (1990) CHUN-CHIEH WUANDHSIU-JU CHENG Department of Atmospheric Sciences, National Taiwan University, Taipei, Taiwan (Manuscript received 27 July 1998, in ®nal form 22 December 1998) ABSTRACT The European Centre for Medium-Range Weather Forecasts Tropical Ocean±Global Atmosphere advanced analysis was used to study the mechanisms that affect the intensity of Typhoons Flo (1990) and Gene (1990). The out¯ow structure, eddy momentum ¯ux convergence, and the mean vertical wind shear were examined. The evolution of potential vorticity (PV) in the out¯ow layer showed low PV areas on top of both Typhoons Flo and Gene, and the low PV areas expanded as the typhoons intensi®ed. The out¯ow pattern of the two typhoons was in¯uenced by the upper-tropospheric environmental systems. The upper-level environmental fea- tures were shown to play a crucial role in the intensi®cation of the two typhoons. The tropical upper-tropospheric trough cell east of Flo provided the out¯ow channel for the typhoon. The enhanced out¯ow, the upper-level eddy ¯ux convergence (EFC), the low vertical wind shear, and the warm sea surface temperature provided all favorable conditions for the development of Flo. On the other hand, the intensi®cation of Gene was associated with its interaction with an upper-level midlatitude trough. The approach of the trough produced upper-level EFC of angular momentum outside 108 lat radius, and the EFC shifted inward with time. -
Significant Data on Major Disasters Worldwide, 1900-Present
DISASTER HISTORY Signi ficant Data on Major Disasters Worldwide, 1900 - Present Prepared for the Office of U.S. Foreign Disaster Assistance Agency for International Developnent Washington, D.C. 20523 Labat-Anderson Incorporated Arlington, Virginia 22201 Under Contract AID/PDC-0000-C-00-8153 INTRODUCTION The OFDA Disaster History provides information on major disasters uhich have occurred around the world since 1900. Informtion is mare complete on events since 1964 - the year the Office of Fore8jn Disaster Assistance was created - and includes details on all disasters to nhich the Office responded with assistance. No records are kept on disasters uhich occurred within the United States and its territories.* All OFDA 'declared' disasters are included - i.e., all those in uhich the Chief of the U.S. Diplmtic Mission in an affected country determined that a disaster exfsted uhich warranted U.S. govermnt response. OFDA is charged with responsibility for coordinating all USG foreign disaster relief. Significant anon-declared' disasters are also included in the History based on the following criteria: o Earthquake and volcano disasters are included if tbe mmber of people killed is at least six, or the total nmber uilled and injured is 25 or more, or at least 1,000 people art affect&, or damage is $1 million or more. o mather disasters except draught (flood, storm, cyclone, typhoon, landslide, heat wave, cold wave, etc.) are included if the drof people killed and injured totals at least 50, or 1,000 or mre are homeless or affected, or damage Is at least S1 mi 1l ion. o Drought disasters are included if the nunber affected is substantial. -
Republic of the Philippines Data Collection Survey on the Incentive Mechanism for Improving Disaster Resiliency of Electric Power Distribution Network Final Report
Republic of the Philippines National Electrification Administration (NEA) Republic of the Philippines Data Collection Survey on the Incentive Mechanism for Improving Disaster Resiliency of Electric Power Distribution Network Final Report October 2015 Japan International Cooperation Agency (JICA) Shikoku Electric Power Co., Inc. (YONDEN) The Japan Economic Research Institute (JERI) 1R JR 15-053 Data Collection Survey on the Incentive Mechanism for Improving Disaster Resiliency of Electric Power Distribution Network: Final Report Contents Chapter 1 Situation of the Energy Sector ............................................................................................ 1 1.1 Economic Conditions in the Philippines .................................................................................... 1 1.2 Current Situation of the Energy Sector in the Philippines.......................................................... 3 1.3 Natural Disaster in the Philippines – Type and Impact .............................................................. 5 Chapter 2 Current Condition of the Power Network in the Philippines ............................................. 8 2.1 Response Organization at Disaster ............................................................................................. 8 2.2 EC Damage and Response Status Information Gathering ........................................................ 14 2.3 ECs’ Capital Investment Plans ................................................................................................. 21 2.4 Financing -
Tropical Cyclones in 1990
ROYAL OBSERVATORY HONG KONG TROPICAL CYCLONES IN 1990 CROWN COPYRIGHT RESERVED Published February 1992 Prepared by Royal Observatory 134A Nathan Road Kowloon Hong Kong Permission to reproduce any part of this publication should be obtained through the Royal Observatory This publication is prepared and disseminated in the interest of promoting the exchange of information. The Government of Hong Kong (including its servants and agents) makes no warranty, statement or representation, expressed or implied, with respect to the accuracy, completeness, or usefulness of the information contained herein, and in so far as permitted by law, shall not have any legal liability or responsibility (including liability for negligence) for any loss, damage or injury (including death) which may result whether directly or indirectly, from the supply or use of such information. This publication is available from: Government Publications Centre General Post Office Building Ground Floor Connaught Place Hong Kong 551.515.2:551.506.1 (512.317) 3 CONTENTS Page FRONTISPIECE: Tracks of tropical cyclones in the western North Pacific and the South China Sea in 1990 FIGURES 4 TABLES 5 HONG KONG'S TROPICAL CYCLONE WARNING SIGNALS 6 1. INTRODUCTION 7 2. TROPICAL CYCLONE SUMMARIES FOR 1990 10 3. REPORTS ON TROPICAL CYCLONES AFFECTING HONG KONG IN 1990 18 (a) Typhoon Marian (9003): 15-19 May 18 (b) Severe Tropical Storm Nathan (9004): 15-19 June 22 (c) Typhoon Percy (9006): 21-30 June 26 (d) Severe Tropical Storm Tasha (9009): 27-31 July 32 (e) Typhoon Becky (9016): 25-30 August 37 (f) Typhoon Ed (9018): 10-20 September 41 4. -
Commander 1N Chief Pacific Com a Nd History
COMMANDER 1N CHIEF PACIFIC COM A ND HISTORY VO 1993 Prepared by the Command History Dirision Office of the Joint Secretary Headquarters USCINCPAC CAMP H.M. SMITH, HAWAII 96861-5028 1995 FC-LIASSIFIED tr,F,cfra,sin by: ,ed Data DIassifled lb tL ; V Authority: SEC 3.1 EQ. 12958 COPY 7OF 60 COPIES SECF1E. COMMANDER IN CRIER PACIFIC COMMAND (USCINCPAC) CAMP H.M. SMITH, HAWAII 96861-4028 J044 5757 Ser S102 31 March 1995 ET Unclassified upon removal of enclosures Subj: PROMULGATION OF USCINCPAC COMMAND HISTORY 1993 Encl: (1) Volume I, USCINCPAC Command History 1993 (2) Volume II, USCINCPAC Command History 1993 1. The USCINCPAC Command History is promulgated in response to the direction of the Joint Chiefs of Staff. 2. This document contains information affecting the security of the United States within the meaning of the Espionage Laws, Title 18, U.S. Code, Sections 793, 794, and 798, and relating to the national defense within the meaning of the Espionage Law, Title 10, U.S. Code, Section 906a. Transmission or reve- lation of its contents in any manner to an unauthorized person is prohibited by law. The classified material contained within this document is to be treated with the utmost discre- tion. Under no circumstances may this information be revealed to anyone other than those whose duties specifically require it. 3. The security classification indicated for each page is according to the highest classification of any portion of it. In those instances when the reverse side of a page is inten- tionally left blank, this is indicated on the preceding page. -
Special Issue on Severe Weather About the Cover
HKMetS BULLETIN, Volume 4, Number 1, 1994 HONG KONG METEOROLOGICAL SOCIETY Special Issue on Severe Weather About the cover The cover picture shows a GMS4-IR image of Typhoon Ira, then a tropical storm, making landfall near Yangjiang at 1500Z on 4 November, 1993. During the next several hours Ira brought heavy rainstorms, particularly over the west of the territory. More details on this and other severe rainfall events which affected Hong Kong in 1993 can be found in several articles in this issue. The Hong Kong Meteorological Society EDITOR-in-CHIEF Bulletin is the official organ of the Society, devoted to editorials, articles, Bill Kyle news, activities and announcements of the Society. EDITORIAL BOARD Members are encouraged to send any articles, media items or information for Johnny C.L. Chan publication in the Bulletin. Y.K. Chan WL. Chang For guidance see the "INFORMATION Edwin S. T. Lai FOR CONTRIBUTORS" in the inside back cover. The Bulletin is copyright. Views expressed in articles or correspondence are those of the Permission to use figures, tables, and authors and do not necessarily brief extracts from this publication in represent those of the Society. any scientific and educational work is hereby granted provided that the source The mention of specific products is properly acknowledged. Any other or companies does not imply any use of the material requires the prior endorsement by the Hong Kong written permission of the Hong Kong Meteorological Society or its Meteorological Society. office bearers in preference to others which are not mentioned. Published by The Hong Kong Meteorological Society c/o Royal Observatory, 134A Nathan Road, Kowloon, Hong Kong. -
4.7. Predicting Tropical Cyclone Intensification
The Association of Tall Eyewall Convection with Tropical Cyclone Intensification A dissertation submitted in partial fulfillment of the requirements for the degree of Doctor of Philosophy at George Mason University By Owen A. Kelley Master of Science George Mason University, 1997 Bachelor of Arts St. John's College, 1993 Director: Michael Summers, Professor Department of Physics and Astronomy Spring Semester 2008 George Mason University Fairfax, VA Copyright 2008 Owen A. Kelley ii ACKNOWLEDGEMENTS Over the past four years, a number of people have discussed with me the ideas in this dissertation. They include Tom Bell, Natalie Blades, Craig Bohren, Scott Braun, Daniel Cecil, Jeff Halverson, Bart Kelley, Genevieve Demos Kelley, John Kwiatkowski, Chris Landsea, Chuntao Liu, David Marks, Daniel Melendez, Mike McCumber, Bob Meneghini, Bill Olson, Dave Silberstein, Joanne Simpson, Erich Stocker, John Stout, and Ed Zipser. The above mentioned people do not necessarily agree with the conclusions of this dissertation, nor are they responsible for any errors. Section 3.9 contains unpublished research from a collaboration with John Stout. The TRMM Science Data and Information System (TSDIS) provided computational facilities. The dissertation was strengthened by suggestions from my adviser Michael Summers and my dissertation committee, which includes Jim Beall, Jeff Halverson, and Menas Kafatos. Various organizations and individuals provided the data used in this dissertation. NASA/JAXA/NICT provided TRMM data. Many TRMM subsets were downloaded from the JAXA Tropical Cyclone Database. NCDC provided ground radar data. The National Hurricane Center (NHC) and the Joint Typhoon Warning Center (JTWC) provided world-wide best track wind intensity estimates. Scott Braun provided output from his simulation of Hurricane Bonnie (1998). -
A Stamping Utility for PDF Documents
Verification of precipitation calculation method based on parameterization of distribution function evolution. I.V.Akimov Hydrometeorological Centre of Russia 11-13 Bolshoy Predtechensky per., 123242, Moscow-242, Russia. E-mail: [email protected] The new precipitation calculation method presented in [1] was evaluated. The method is based on different approach, than used in Kessler-type parameterizations. In Kessler approach [2] the precipitation beginning from cloudy layer is permitted after cloud water content reaches some value, named as autoconversion threshold. But the value of this threshold could not be obtained from the measurements and the hypothesis about such threshold contradicts with the observed facts that precipitation starts from clouds when cloud water content rises up to quite different values [3,4]. Therefore the new method is based on consideration of precipitation beginning process as formation of part of cloud spectra consisting of large drops which falls out as a precipitation. This is realized by parametric description distribution function evolution during precipitation formation process. The main processes that influence on distribution function evolution are gravitational and turbulent coalescence in liquid clouds and sublimation of water vapour on ice crystals in mixed phase clouds. The proposed method was included as precipitation parameterization module in global spectral model of the Hydrometcentre of Russia T85L31. The precipitation calculation module that was used in old version of model is based on Kessler parameterization. Therefore the comparison of precipitation fields obtained from new version of model with results obtained from old version allows to evaluate the distinction between two different parameterization approaches. Two scores were chosen for verification analysis.