Cellular Senescence, Cancer and Aging: the Telomere Connection
Total Page:16
File Type:pdf, Size:1020Kb
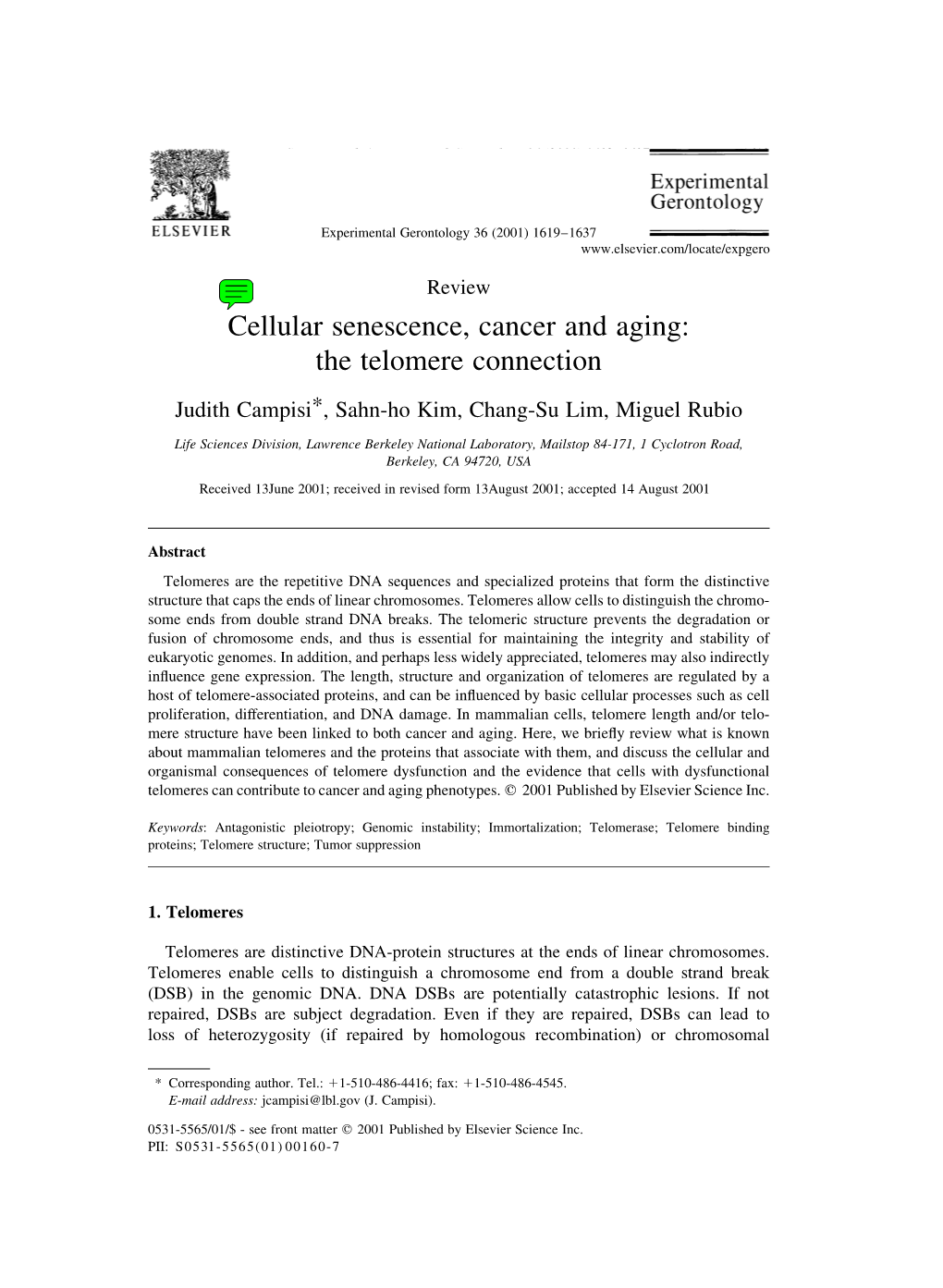
Load more
Recommended publications
-
Cellular Senescence Promotes Skin Carcinogenesis Through P38mapk and P44/P42mapk Signaling
Author Manuscript Published OnlineFirst on July 8, 2020; DOI: 10.1158/0008-5472.CAN-20-0108 Author manuscripts have been peer reviewed and accepted for publication but have not yet been edited. Cellular senescence promotes skin carcinogenesis through p38MAPK and p44/p42 MAPK signaling Fatouma Alimirah1, Tanya Pulido1, Alexis Valdovinos1, Sena Alptekin1,2, Emily Chang1, Elijah Jones1, Diego A. Diaz1, Jose Flores1, Michael C. Velarde1,3, Marco Demaria1,4, Albert R. Davalos1, Christopher D. Wiley1, Chandani Limbad1, Pierre-Yves Desprez1, and Judith Campisi1,5* 1Buck Institute for Research on Aging, Novato, CA 94945, USA 2Dokuz Eylul University School of Medicine, Izmir 35340, Turkey 3Institute of Biology, University of the Philippines Diliman, College of Science, Quezon City 1101, Philippines 4European Research Institute for the Biology of Ageing, University Medical Center Groningen, Groningen, the Netherlands 5Biosciences Division, Lawrence Berkeley National Laboratory, Berkeley, CA 94720, USA *Lead Contact: Judith Campisi, Buck Institute for Research on Aging, 8001 Redwood Boulevard, Novato, CA 94945, USA; Email: [email protected] or [email protected]. Telephone: 1-415-209-2066; Fax: 1-415-493-3640 Running title: Senescence and skin carcinogenesis Key words: doxorubicin, senescence-associated secretory phenotype, squamous cell carcinoma, microenvironment, inflammation, transgenic mice, tumorspheres Conflict of Interests JC is a co-founder of Unity Biotechnology and MD owns equity in Unity Biotechnology. All other authors declare no competing financial interests. Downloaded from cancerres.aacrjournals.org on September 25, 2021. © 2020 American Association for Cancer Research. Author Manuscript Published OnlineFirst on July 8, 2020; DOI: 10.1158/0008-5472.CAN-20-0108 Author manuscripts have been peer reviewed and accepted for publication but have not yet been edited. -
Cellular Senescence and Apoptosis: How Cellular Responses Might Influence Aging Phenotypes
Experimental Gerontology 38 (2003) 5–11 www.elsevier.com/locate/expgero Cellular senescence and apoptosis: how cellular responses might influence aging phenotypes Judith Campisia,b,* aLife Sciences Division, Lawrence Berkeley National Laboratory, 1 Cyclotron Road, Berkeley, CA 94720, USA bBuck Institute for Age Research, 8001 Redwood Blvd., Novato, CA 94945, USA Received 20 May 2002; accepted 28 May 2002 Abstract Aging in complex multi-cellular organisms such as mammals entails distinctive changes in cells and molecules that ultimately compromise the fitness of adult organisms. These cellular and molecular changes lead to the phenotypes we recognize as aging. This review discusses some of the cellular and molecular changes that occur with age, specifically changes that occur as a result of cellular responses that evolved to ameliorate the inevitable damage that is caused by endogenous and environmental insults. Because the force of natural selection declines with age, it is likely that these processes were never optimized during their evolution to benefit old organisms. That is, some age- related changes may be the result of gene activities that were selected for their beneficial effects in young organisms, but the same gene activities may have unselected, deleterious effects in old organisms, a phenomenon termed antagonistic pleiotropy. Two cellular processes, apoptosis and cellular senescence, may be examples of antagonistic pleiotropy. Both processes are essential for the viability and fitness of young organisms, but may contribute to aging phenotypes, including certain age-related diseases. q 2002 Elsevier Science Inc. All rights reserved. Keywords: Antagonistic pleiotropy; Apoptosis; Cancer; Cellular senescence; DNA damage response; Neurodegeneration; p53; Telomeres 1. -
Cellular Senescence and the Senescent Secretory Phenotype: Therapeutic Opportunities
Cellular senescence and the senescent secretory phenotype: therapeutic opportunities Tamara Tchkonia, … , Judith Campisi, James L. Kirkland J Clin Invest. 2013;123(3):966-972. https://doi.org/10.1172/JCI64098. Review Series Aging is the largest risk factor for most chronic diseases, which account for the majority of morbidity and health care expenditures in developed nations. New findings suggest that aging is a modifiable risk factor, and it may be feasible to delay age-related diseases as a group by modulating fundamental aging mechanisms. One such mechanism is cellular senescence, which can cause chronic inflammation through the senescence-associated secretory phenotype (SASP). We review the mechanisms that induce senescence and the SASP, their associations with chronic disease and frailty, therapeutic opportunities based on targeting senescent cells and the SASP, and potential paths to developing clinical interventions. Find the latest version: https://jci.me/64098/pdf Review series Cellular senescence and the senescent secretory phenotype: therapeutic opportunities Tamara Tchkonia,1 Yi Zhu,1 Jan van Deursen,1 Judith Campisi,2 and James L. Kirkland1 1Robert and Arlene Kogod Center on Aging, Mayo Clinic, Rochester, Minnesota, USA. 2Buck Institute for Research on Aging, Novato, California, USA. Aging is the largest risk factor for most chronic diseases, which account for the majority of morbidity and health care expenditures in developed nations. New findings suggest that aging is a modifiable risk factor, and it may be feasible to delay age-related diseases as a group by modulating fundamental aging mechanisms. One such mech- anism is cellular senescence, which can cause chronic inflammation through the senescence-associated secretory phenotype (SASP). -
Revisiting the Hayflick Limit
bioRxiv preprint doi: https://doi.org/10.1101/2021.05.03.442497; this version posted May 4, 2021. The copyright holder for this preprint (which was not certified by peer review) is the author/funder. All rights reserved. No reuse allowed without permission. Revisiting the Hayflick Limit: Insights from an Integrated Analysis of Changing Transcripts, Proteins, Metabolites and Chromatin. Michelle Chan, Han Yuan*, Ilya Soifer*, Tobias M. Maile, Rebecca Y. Wang, Andrea Ireland, Jonathon O’Brien, Jérôme Goudeau, Leanne Chan, Twaritha Vijay, Adam Freund, Cynthia Kenyon, Bryson Bennett, Fiona McAllister, David R. Kelley, Margaret Roy, Robert L. Cohen, Arthur D. Levinson, David Botstein**, David G. Hendrickson** *contributed equally **Corresponding Authors Abstract Replicative senescence (RS) as a model has become the central focus of research into cellular aging in vitro. Despite decades of study, this process through which cells cease dividing is not fully understood in culture, and even much less so in vivo during development and with aging. Here, we revisit Hayflick’s original observation of RS in WI-38 human fetal lung fibroblasts equipped with a battery of high dimensional modern techniques and analytical methods to deeply profile the process of RS across each aspect of the central dogma and beyond. We applied and integrated RNA-seq, proteomics, metabolomics, and ATAC-seq to a high resolution RS time course. We found that the transcriptional changes that underlie RS manifest early, gradually increase, and correspond to a concomitant global increase in accessibility in nucleolar and lamin associated domains. During RS WI-38 fibroblast gene expression patterns acquire a striking resemblance to those of myofibroblasts in a process similar to the epithelial to mesenchymal transition (EMT). -
Judith Campisi, Publications Original Research Articles Suzuki H
Judith Campisi, Publications Original Research Articles Suzuki H, Nishimura Y, Iketani H, Campisi J, Hirashima A, Inouye M, Hirota Y. 1976. A novel mutation that causes a structural change in a lipoprotein in the outer membrane of Escherichia coli.. J Bact 127:494-2501. Campisi J, Scandella CJ. 1978. Fertilization-induced changes in membrane fluidity of sea urchin eggs. Science 199:1336-1337. Campisi J, Scandella CJ. 1980. Bulk membrane fluidity increases after fertilization and partial activation of sea urchin eggs. J Biol Chem 255:5411-5419. Campisi J, Scandella CJ. 1980. Calcium induces a decrease in membrane fluidity of the sea urchin cortex after fertilization. Nature 286:185-186. Campisi J, Pardee AB. 1981. Cellular mutations and drug resistance probed by herpes simplex virus. J Cell Physiol 109:469-480. Campisi J, Medrano EE, Morreo G, Pardee AB. 1982. Restriction point control of cell growth by a labile protein: Evidence for increased stability in transformed cells. Proc Natl Acad Sci USA 79:436-440. Campisi J, Pardee AB. 1982. An artifact in measurement of S phase initiation and its implications for the kinetics of S phase-specific enzyme activities. Exp Cell Res 140:389-393. Campisi J, Hafner J, Boorstein RB, Pardee AB 1983. Hereditary orotic aciduria, xeroderma pigmentosum and Lesch-Nyhan syndrome probed by herpes simplex virus: 125I-- Iododeoxycytidine incorporation as an assay for viral growth. J Cell Physiol 114:21-28. Campisi J, Medrano EE. 1983. Cell cycle perturbations in normal and transformed cells caused by detachment from the substratum. J Cell Physiol 114:53-60. Boorstein R, Campisi J, Pardee AB. -
The Thorny Path Linking Cellular Senescence to Organismal Aging Christopher K
Mechanisms of Ageing and Development 126 (2005) 1040–1045 www.elsevier.com/locate/mechagedev Progress paper The thorny path linking cellular senescence to organismal aging Christopher K. Patil a, I. Saira Mian a, Judith Campisi a,b,* a Life Sciences Division, Lawrence Berkeley National Laboratory, 1 Cyclotron Road, Berkeley, CA 94720, USA b Buck Institute for Age Research, 8001 Redwood Boulevard, Novato, CA 94545, USA Accepted 12 August 2005 Keywords: Cellular senescence; Telomeres; Tumor suppression; Stress resistance Half a century is fast approaching since Hayflick and 1. At least two pathways limit replicative capacity colleagues formally described the limited ability of normal human cells to proliferate in culture (Hayflick and Moor- Since Hayflick’s seminal observations, much progress has head, 1961). This finding – that normal somatic cells, in been made in understanding why many cells do not undergo contrast to cancer cells, cannot divide indefinitely – proliferation (used here interchangeably with growth) challenged the prevailing idea that cells from mortal indefinitely in culture. We now understand, at least broadly, multicellular organisms were intrinsically ‘immortal’ that replicative capacity is limited by two pathways—one (Carrell, 1912). It also spawned two hypotheses, essential induced by telomere erosion and genotoxic stress, and another elements of which persist today. The first held that the induced by stress of an unknown nature. Moreover, other as restricted proliferation of normal cells, now termed cellular yet unidentified pathways may also restrict cell division. senescence, suppresses cancer (Hayflick, 1965; Sager, 1991; Campisi, 2001). The second hypothesis, as explained in the article by Lorenzini et al., suggested that the limited 2. -
Cellular Senescence: When Bad Things Happen to Good Cells
REVIEWS Cellular senescence: when bad things happen to good cells Judith Campisi* and Fabrizio d’Adda di Fagagna‡ Abstract | Cells continually experience stress and damage from exogenous and endogenous sources, and their responses range from complete recovery to cell death. Proliferating cells can initiate an additional response by adopting a state of permanent cell-cycle arrest that is termed cellular senescence. Understanding the causes and consequences of cellular senescence has provided novel insights into how cells react to stress, especially genotoxic stress, and how this cellular response can affect complex organismal processes such as the development of cancer and ageing. Renewable tissue Cellular senescence was formally described more than of cellular senescence, and the evidence that it is impor- A tissue in which cell four decades ago when Hayflick and colleagues showed tant for suppressing cancer and a possible contributor proliferation is important for that normal cells had a limited ability to proliferate in to ageing. tissue repair or regeneration. culture1 (see BOX 1 for descriptions of different types Renewable tissues typically ) Senescence in an evolutionary context contain, but sometimes recruit, of senescence . These classic experiments showed that mitotic cells upon injury or cell human fibroblasts initially underwent robust cell division To understand how cellular senescence can be both bene- loss. in culture. However, gradually — over many cell doub- ficial and detrimental, and the origins of its regulation, lings — cell proliferation (used here interchangeably it is important to understand the nature of cancer and with cell growth) declined. Eventually, all cells in the the evolutionary theory of ageing. Cancer is often fatal culture lost the ability to divide. -
Cancer and Ageing: Rival Demons?
REVIEWS CANCER AND AGEING: RIVAL DEMONS? Judith Campisi Organisms with renewable tissues use a network of genetic pathways and cellular responses to prevent cancer. The main mammalian tumour-suppressor pathways evolved from ancient mechanisms that, in simple post-mitotic organisms, act predominantly to regulate embryogenesis or to protect the germline. The shift from developmental and/or germline maintenance in simple organisms to somatic maintenance in complex organisms might have evolved at a cost. Recent evidence indicates that some mammalian tumour-suppressor mechanisms contribute to ageing. How might this have happened, and what are its implications for our ability to control cancer and ageing? COMPLEX ORGANISMS Cancer afflicts COMPLEX ORGANISMS, such as mice and have been studied extensively, primarily in mice and Multicellular organisms that are humans, almost inevitably as they approach middle and humans. It is clear that at least two main strategies composed of both post-mitotic old age1–3 (FIG. 1; BOX 1). This is not the case for all organ- evolved to suppress cancer4. One mechanism uses and renewable (mitotic) somatic isms, however; the SIMPLE model invertebrate organisms CARETAKER proteins to protect the genome from acquiring tissues. Caenorhabditis elegans and Drosophila melanogaster,for potentially oncogenic mutations. The other uses SIMPLE ORGANISMS example, do not develop cancer. So, how prevalent is can- GATEKEEPER proteins to eliminate or prevent the growth of Multicellular organisms that are cer among animals, and what distinguishes organisms potential cancer cells (FIG. 2). Both mechanisms evolved composed entirely or largely of that develop cancer from those that do not? from ancestral genetic pathways, elements of which were, post-mitotic somatic cells. -
From Ancient Pathways to Aging Cells-Connecting Metabolism And
Cell Metabolism Review From Ancient Pathways to Aging Cells— Connecting Metabolism and Cellular Senescence Christopher D. Wiley1,* and Judith Campisi1,2,* 1Buck Institute for Research on Aging, 8001 Redwood Boulevard, Novato, CA 94945, USA 2Lawrence Berkeley National Laboratory, 1 Cyclotron Road, Berkeley, CA 94720, USA *Correspondence: [email protected] (C.D.W.), [email protected] (J.C.) http://dx.doi.org/10.1016/j.cmet.2016.05.010 Cellular senescence is a complex stress response that permanently arrests the proliferation of cells at risk for oncogenic transformation. However, senescent cells can also drive phenotypes associated with aging. Although the senescence-associated growth arrest prevents the development of cancer, and the metabolism of cancer cells has been studied in depth, the metabolic causes and consequences of cellular senescence were largely unexplored until recently. New findings reveal key roles for several aspects of cellular meta- bolism in the establishment and control of senescent phenotypes. These discoveries have important impli- cations for both cancer and aging. In this review, we highlight some of the recent links between metabolism and phenotypes that are commonly associated with senescent cells. Introduction studies and their relevance within the larger framework of cancer Cellular senescence is a multifaceted process that radically and aging. alters the phenotype of metazoan cells that have the ability to divide. Two hallmarks of cellular senescence are an essentially General Metabolic Features of Senescent Cells irreversible arrest of cell proliferation and the development of a Decades ago, Otto Warburg observed that cancer cells generally multi-component senescence-associated secretory phenotype differ from normal cells by the manner in which they metabolize (SASP). -
Replicative Senescence: Minireview an Old Lives' Tale?
CORE Metadata, citation and similar papers at core.ac.uk Provided by Elsevier - Publisher Connector Cell, Vol. 84, 497±500, February 23, 1996, Copyright 1996 by Cell Press Replicative Senescence: Minireview An Old Lives' Tale? Judith Campisi underlying cause of organismic aging. This idea is much Life Sciences Division more controversial and less intuitively obvious. How did Department of Cancer Biology it come about, and what is the evidence for it? Berkeley National Laboratory University of California Berkeley, California 94720 All Men Are Mortal (but What of Their Cells?) The observation that cell division is inherently limited Normal animal cells, with few exceptions, do not divide contradicted an earlier belief, championed by Carrel, indefinitely. This property, termed the finite replicative that vertebrate cells can proliferate indefinitely once re- life span of cells, leads to an eventual arrest of cell moved from the organism. Carrel's belief stemmed in division by a process termed cellular or replicative se- part from his apparent (but since irreproducible) ability nescence. continually to subculture chick cells. This study and Although predicted and observed earlier, replicative others (see Hayflick, 1965) spawned the idea that cells senescence was first formally described over 30 years may be intrinsically ªimmortal.º Implicit in some of these ago when Hayflick and his colleagues reported that hu- studies was the idea that understanding cell immortality man fibroblasts gradually and inevitably lost their ability might uncover the basis for organismic mortality (Carrel, to proliferate upon continual subculture (Hayflick, 1965). 1912). With this idea as a backdrop, then, Hayflick as- Since then, many cell types from many animal species serted that ªnormal human diploid cell strains in vitro have been shown to have a finite replicative life span are in fact `mortal'º and suggested that replicative life (see Stanulis-Praeger, 1987). -
SENS Research Foundation Annual Report 2012
annual report april 2013 contents Introduction . 2 Research Summary . 3-10 Outreach . 11-14 Education . 15-16 Finances . 17-18 sens research foundation - 110 Pioneer Way, Suite J - Mountain View, CA 94041 - USA phone: 650-336-1780 - fax: 650-336-1781 - www.sens.org Chief Executive Officer Board of Directors © 2013, SENS Research Foundation Mike Kope Jonathan Cain All rights reserved. Kevin Dewalt Chief Science Officer Bill Liao No part of this material may be reproduced without specific Aubrey de Grey Barbara Logan permission from SENS Research Foundation. James O’Neill Chief Operating Officer Kevin Perrott SENS Research Foundation’s federal employer ID number is 94-3473864. Because SENS Research Foundation is a 501(c)(3) Tanya Jones reimagine Mike Kope non-profit, your donation may be tax deductible. A world free of age-related disease “The biggest driver of our long-term debt is the rising cost of healthcare for an aging population.” – President Barack Obama’s 2013 State of the Union address1 . The United States will spend more than one trillion dollars There is only one charity in the world doing the work – on healthcare for seniors this year 2. Heart disease, the scientific, educational, and outreach-based – to address greatest of the US’s health problems, has recently become those causes . the developing world’s biggest killer as well .3 The economic crisis noted in the President’s decisive comment is not Please, take a moment and reimagine aging with us . ours alone: it is everyone’s . Meanwhile, the worldwide suffering caused by the diseases of aging remains too Start by imagining a cure for cardiovascular disease 5. -
Cellular Senescence: Putting the Paradoxes in Perspective Judith Campisi1,2
This article appeared in a journal published by Elsevier. The attached copy is furnished to the author for internal non-commercial research and education use, including for instruction at the authors institution and sharing with colleagues. Other uses, including reproduction and distribution, or selling or licensing copies, or posting to personal, institutional or third party websites are prohibited. In most cases authors are permitted to post their version of the article (e.g. in Word or Tex form) to their personal website or institutional repository. Authors requiring further information regarding Elsevier’s archiving and manuscript policies are encouraged to visit: http://www.elsevier.com/copyright Author's personal copy Available online at www.sciencedirect.com Cellular senescence: putting the paradoxes in perspective Judith Campisi1,2 Cellular senescence arrests the proliferation of potential cancer Under some circumstances, senescent cells can promote cells, and so is a potent tumor suppressive mechanism, akin to tumor progression, in obvious and apparent contradiction apoptosis. Or is it? Why did cells evolve an anti-cancer to its being tumor suppressive. And under other circum- mechanism that arrests, rather than kills, would-be tumor cells? stances, senescent cells appear to aid tissue repair. Emer- Recent discoveries that senescent cells secrete growth factors, ging data now provide a framework for understanding proteases and cytokines provide a shifting view—from the strikingly different consequences of cellular senes- senescence as a cell autonomous suppressor of tumorigenesis cence, and suggest strategies for harnessing the benefits to senescence as a means to mobilize the systemic and local of this process, while mitigating its potentially deleterious tissue milieu for repair.