Ultrashort Pulse Characterisation Using an Acousto-Optic Bulk Pulse Shaper
Total Page:16
File Type:pdf, Size:1020Kb
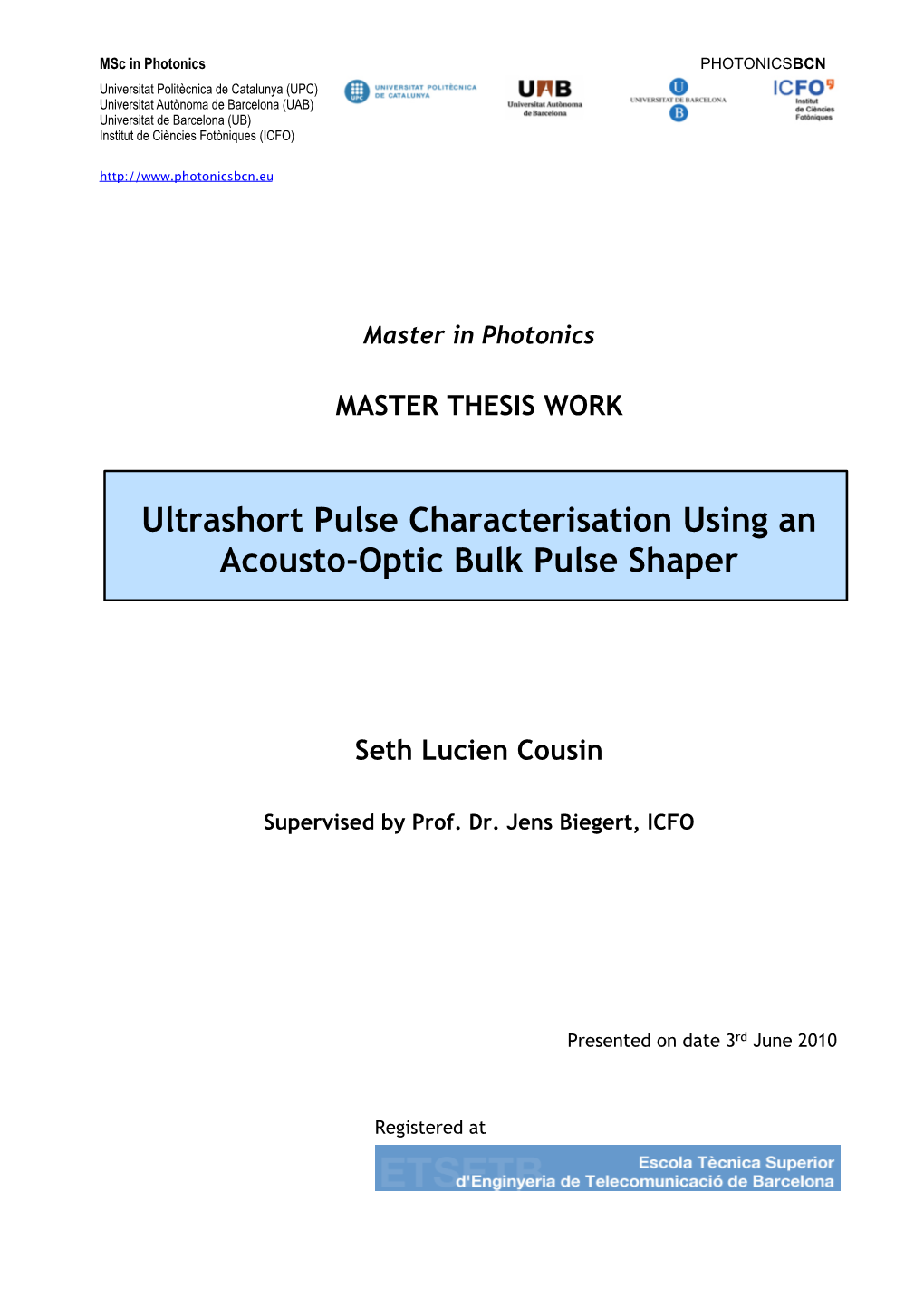
Load more
Recommended publications
-
Blazing Through the Darkness
Blazing through the darkness MICROFIRE PRODUCT CATALOG - 2019 All products can be custom made to your specific needs www.microfire-system.com MICROFIRE CATALOG - 2019 1 MicroFire is a leading technology company with over 10 years of experience in designing and manufacturing top-of-the-line tactical lighting Table of Contents products including flashlights, searchlights, and laser dazzlers. Driven by innovation, we at MicroFire are constantly looking for new ways to 1 Company Profile…….…….….…….1 translate cutting-edge technology into value, 2 Product Information.……….........2 quality, and ultimately superior performance. All of our products are made per U.S. MIL-SPEC 2.1 Hand-held Flashlights.………..2 and we pride ourselves in our mission to provide 2.2 Weapon Lights…………………..15 combat and law enforcement personnel, including some of the most elite troops around 2.3 Searchlights……………………...28 the world, with the best lighting solutions possible. 2019 marks a another beginning for us. With the successful introduction of several ground- breaking products that have surpassed all major competitors last year, MicroFire is now poised to transform the landscape of the entire tactical lighting industry. MICROFIRE CATALOG - 2019 2 Hand-held Tactical Flashlights Our relentless pursuit for excellence and perfection pushes the boundaries of what a flashlight can do. Although no single flashlight is suitable for all circumstances, when you look for a lighting product to fulfill your tactical need, look no further than MicroFire: you are guaranteed a top-quality, ultra-reliable and most durable product that can help you complete the mission successfully and return safely. At MicroFire there is always a lighting product that can meet or even exceed your expectations. -
Tactical Employment of Nonlethal Weapons
NLW MULTI-SERVICE TACTICS, TECHNIQUES, AND PROCEDURES FOR THE TACTICAL EMPLOYMENT OF NONLETHAL WEAPONS FM 3-22.40 MCWP 3-15.8 NTTP 3-07.3.2 AFTTP(I) 3-2.45 October 2007 DISTRIBUTION RESTRICTION: Distribution authorized to US Government agencies and their contractors only to protect technical or operational information from automatic dissemination under the International Exchange Program or by other means. This determination was made on 15 Nov 2006. Other requests will be referred to: HQ TRADOC, ATTN: ATFC-EJ, Ft Monroe, VA 23651-1067; HQ MCCDC, ATTN: C427, Quantico, VA 22134-5021; NWDC, ATTN: N5, Newport, RI 02841-1207; or AFDDEC, ATTN: DD, Maxwell AFB, 36112-6112. DESTRUCTION NOTICE: Destroy by any method that will prevent disclosure of contents or reconstruction of the document. This publication contains copyright material. See figure III-6. FOREWORD This publication has been prepared under our direction for use by our respective commands and other commands as appropriate. BARBARA G. FAST ANDREW W. O’DONNELL, JR. Major General, US Army Brigadier General (Sel), US Marine Corps Deputy Director/Chief of Staff, Director Army Capabilities Integration Center Capabilities Development Directorate CARLTON B. JEWETT ALLEN G. PECK Rear Admiral, US Navy Major General, US Air Force Commander Commander Navy Warfare Development Command Air Force Doctrine Development and Education Center This publication is available through the ALSA Web site (www.alsa.mil); through the Army at Army Knowledge Online (AKO) (www.us.army.mil) and at the General Dennis J. Reimer Training and Doctrine Digital Library (www.train.army.mil) Web sites; and through the Air Force at the Air Force Publishing Web site (www.e-publishing.af.mil). -
Laser Dazzler
Application Notes Compact Optics Module— For remote laser dazzler Introduction The portable laser dazzlers are tested with a precise Laser dazzler is a new type of non-lethal weapon, to eye safe power density for mere TEMPORARY vision temporarily disable its target with flash blindness. impairment device. They provide the following Targets can include sensors or human vision. In addition advantages: to military use, laser dazzler is now widely employed in • Diversification: Using optical modulation, effective civil applications, such as security. laser beam expansion can be realized to control the power density. An external focusing lens is used to Operation Principle control the beam size. The light intensity and spot The dazzler uses a green laser as the light source set to 8 can be varied to achieve an effective value in the times strobe per second. This value was chosen to range of 1m-1000m. 50m distance expansion beam resonate with the biological frequency in the human diameter is 25m; 100m spot diameter is 40m; body, causing dizziness. Through optical modulation, the 1000m spot diameter can reach 100m. use of such a laser source results in the eyes suddenly • Intelligence: It can also be remotely and flexibly appearing "laser flash blind”, effectively deterring or maneuvered both horizontally and vertically. subduing criminal suspects remotely without damaging • Convenience: By configuring the digital circuit and the attacked subject. power supply, the device is portable. At the same time, it is equipped with a car charger to support full day use. • Robust: The laser can be fired in any direction at any time. -
Investment Strategy for Science and Technology Outlines Space Technologies
1 Science and Technology on a Mission 3 A Strategy for Science and Technology Investments 7 Director’s Initiatives 7 Predictive Biology 8 Engineering the Carbon Economy 10 Cognitive Simulation 12 Space Science and Security 13 Accelerated Materials and Manufacturing 13 Core Competencies 13 Accelerated Materials and Manufacturing 15 High-Energy-Density Science 19 High-Performance Computing, Simulation, and Data Science 24 Nuclear, Chemical, and Isotopic Science and Technology 27 Lasers and Optical Science and Technology 29 Bioscience and Bioengineering 31 Earth and Atmospheric Science 12/33 Mission Research Challenges 12 Space Science and Security 33 Quantum Science and Technology 34 Nuclear Weapons Science TABLE OF CONTENTS TABLE 36 High Explosive Physics, Chemistry, and Material Science 37 Nuclear Threat Reduction 38 Chemical and Biological Countermeasures 39 Directed Energy 40 Forensic Science 42 Cybersecurity and Cyber–Physical Resilience 43 Energy and Resource Security 45 Hypersonics ii Science and Technology on a Mission Our founders created Lawrence I’d like to highlight three items in our investment approach this Livermore National Laboratory year. First, as a national resource for the U.S., LLNL is using its (LLNL) as a “new ideas” lab, a science and technology capabilities to help the nation respond place where innovative science to the COVID-19 pandemic; second, we are paying enhanced and technical solutions to the attention to the White House-identified technologies of the nation’s most difficult security future; and third, we have added hypersonics science and challenges are created. We technology as a mission research challenge. continue this tradition, living our motto, “Science and Technology In response to the COVID-19 crisis, LLNL has invested on a Mission,” by pushing the LDRD funds, and other investments, to help the nation frontier of what is or might be better understand the SARS-CoV-2 virus and help find scientifically and technically therapeutic solutions. -
Two-Dimensional Electronic Spectroscopy of the Photosystem II D1D2-Cyt.B559 Reaction Center Complex
Two-Dimensional Electronic Spectroscopy of the Photosystem II D1D2-cyt.b559 Reaction Center Complex by Jeffrey Allen Myers A dissertation submitted in partial fulfillment of the requirements for the degree of Doctor of Philosophy (Physics) in The University of Michigan 2010 Doctoral Committee: Assistant Professor Jennifer P. Ogilvie, Chair Professor Paul R. Berman Professor Timothy A. McKay Professor Roseanne J. Sension Assistant Professor Kevin J. Kubarych ⃝c Jeffrey Allen Myers 2010 All Rights Reserved To my parents, Dan and Tina Myers ii ACKNOWLEDGEMENTS My time at the University of Michigan has been spent learning a great deal about myself and the people around me, as well as the intricacies of physics. As an un- dergraduate, I never guessed what it really means to get a Ph.D. It has indeed been an adventure of great scope, a keen expansion of my knowledge and problem-solving capabilities, and a refinement of my identity as a scientist. But to stop there without acknowledging the many, many people who have helped me over the years would be a great injustice. First, I would like to thank everyone in Professor Ogilvie's group, all of whom brought some sunshine into a lab which, despite being on the corner of the fourth floor, has no windows. Dr. Sarah Nichols has my eternal gratitude for lending me her LATEXbook and example code to get me started up the typesetting learning curve, without which I would have been hopelessly lost in formatting. Kristin Lewis has been by my side in the lab from day one as my lab partner and academic counterpart. -
Multispectral Multidimensional Spectrometer Spanning the Ultraviolet to the Mid-Infrared
Multispectral multidimensional spectrometer spanning the ultraviolet to the mid-infrared Cite as: Rev. Sci. Instrum. 90, 013108 (2019); https://doi.org/10.1063/1.5055244 Submitted: 06 September 2018 . Accepted: 29 November 2018 . Published Online: 24 January 2019 Yin Song, Arkaprabha Konar, Riley Sechrist, Ved Prakash Roy, Rong Duan, Jared Dziurgot, Veronica Policht, Yassel Acosta Matutes, Kevin J. Kubarych, and Jennifer P. Ogilvie ARTICLES YOU MAY BE INTERESTED IN Signatures of vibronic coupling in two-dimensional electronic-vibrational and vibrational- electronic spectroscopies The Journal of Chemical Physics 147, 094202 (2017); https://doi.org/10.1063/1.4991745 Theory of coherent two-dimensional vibrational spectroscopy The Journal of Chemical Physics 150, 100901 (2019); https://doi.org/10.1063/1.5083966 Two-dimensional vibrational-electronic spectroscopy The Journal of Chemical Physics 143, 154201 (2015); https://doi.org/10.1063/1.4932983 Rev. Sci. Instrum. 90, 013108 (2019); https://doi.org/10.1063/1.5055244 90, 013108 © 2019 Author(s). Review of ARTICLE Scientific Instruments scitation.org/journal/rsi Multispectral multidimensional spectrometer spanning the ultraviolet to the mid-infrared Cite as: Rev. Sci. Instrum. 90, 013108 (2019); doi: 10.1063/1.5055244 Submitted: 6 September 2018 • Accepted: 29 November 2018 • Published Online: 24 January 2019 Yin Song,1 Arkaprabha Konar,1 Riley Sechrist,1 Ved Prakash Roy,2 Rong Duan,2 Jared Dziurgot,1 Veronica Policht,1 Yassel Acosta Matutes,1 Kevin J. Kubarych,2 and Jennifer P. Ogilvie1,a) AFFILIATIONS 1 Department of Physics, University of Michigan, 450 Church St., Ann Arbor, Michigan 48109, USA 2Department of Chemistry, University of Michigan, 930 N University Ave., Ann Arbor, Michigan 48109, USA a)Author to whom correspondence should be addressed: [email protected] ABSTRACT Multidimensional spectroscopy is the optical analog to nuclear magnetic resonance, probing dynamical processes with ultrafast time resolution. -
Design and Verification of a Multi-Terawatt Ti-Sapphire Femtosecond Laser System
University of Central Florida STARS Electronic Theses and Dissertations, 2004-2019 2017 Design and Verification of a Multi-Terawatt Ti-Sapphire Femtosecond Laser System Patrick Roumayah University of Central Florida Part of the Optics Commons Find similar works at: https://stars.library.ucf.edu/etd University of Central Florida Libraries http://library.ucf.edu This Masters Thesis (Open Access) is brought to you for free and open access by STARS. It has been accepted for inclusion in Electronic Theses and Dissertations, 2004-2019 by an authorized administrator of STARS. For more information, please contact [email protected]. STARS Citation Roumayah, Patrick, "Design and Verification of a Multi-Terawatt Ti-Sapphire Femtosecond Laser System" (2017). Electronic Theses and Dissertations, 2004-2019. 5404. https://stars.library.ucf.edu/etd/5404 DESIGN AND VERIFICATION OF A MULTI-TERAWATT TI-SAPPHIRE FEMTOSECOND LASER SYSTEM by PATRICK ROUMAYAH B.S. University of Michigan, 2011 A thesis submitted in partial fulfillment of the requirements for the degree of Master of Science in the College of Optics and Photonics at the University of Central Florida Orlando, Florida Spring Term 2017 Major Professor: Martin Richardson ABSTRACT Ultrashort pulse lasers are well-established in the scientific community due to the wide range of applications facilitated by their extreme intensities and broad bandwidth capabilities. This thesis will primarily present the design for the Mobile Ultrafast High Energy Laser Facility (MU-HELF) for use in outdoor atmospheric propagation experiments under development at the Laser Plasma Laboratory at UCF. The system is a 100fs 500 mJ Ti-Sapphire Chirped-Pulse Amplification (CPA) laser, operating at 10 Hz. -
Efficient Generation and Characterization of Soft X-Ray By
Efficient generation and characterization of soft x-ray by laser-driven high-order harmonic generation ANNE-LISE VIOTTI Master’s Thesis at Paul Scherrer Institute Supervisor at KTH: Valdas Pasiskevicius Supervisor at PSI: Christoph Hauri Examiner: Fredrik Laurell TRITA-FYS 2014:53 ISSN 0280-316X ISRN KTH/FYS/–14:53–SE Abstract As a final year student specialized in laser physics and nonlinear optics at the Royal Institute of Technology (KTH, Stockholm), I chose to explore the field of high-harmonic generation by doing a master thesis at the Paul Scherrer Institute in Switzerland. There, in the laser group of the SwissFEL project, researchers are developing new table-top laser systems able to generate high-order harmonics in several gas media. The context of this master thesis project is the future scientific large-scale facil- ity under construction at Paul Scherrer Institute (PSI). The SwissFEL project is a x-ray free electron laser which should provide soon new opportunities for the re- search world. The main advantage of this new type of light source is the ability to witness fast processes in detail. Those processes which can be found in nature or in the human body are occurring very rapidly. For instance, this kind of facility could help look- ing for new types of medicine drugs or new types of materials... To develop this unique kind of light facility, PSI’s researchers have gained expe- rience with the SLS facility which is a synchrotron (Swiss Light Source). Among several areas of research, work on proton therapy was carried out in order to deal with certain types of cancer. -
Generation of Few-Cycle Multi-Millijoule 2.5 Μm Pulses from A
www.nature.com/scientificreports OPEN Generation of few-cycle multi- millijoule 2.5 μm pulses from a single-stage Cr2+:ZnSe amplifer Yi Wu1, Fangjie Zhou1, Esben W. Larsen2 ✉ , Fengjiang Zhuang1, Yanchun Yin1 & Zenghu Chang 1 Lasers capable of generating attosecond X-ray pulses in the water window (282 to 533 eV) through high- order harmonic generation are normally based on inefcient, multi-stage optical parametric amplifers or optical parametric chirped pulse amplifers pumped by femtosecond or picosecond lasers. Here we report a very efcient single amplifcation stage laser based on traditional chirped pulse amplifcation capable of producing 4 mJ, near-transform limited 44 fs (<6 cycles), 1 kHz pulses centered at 2.5 μm. The ≈90 GW peak power is the highest value ever reached at this wavelength. In order to fully compress the laser pulses our system is built in a nitrogen box. Our system utilizes water cooled chromium doped zinc selenide (Cr2+:ZnSe) as the gain medium and is pumped by a commercial nanosecond holmium doped yttrium-aluminum-garnet (Ho:YAG) laser. Te natural time scale of electron dynamics in atoms, molecules, and condensed matter is on the order of atto- seconds. Since the frst demonstration of tabletop attosecond light sources from high-order harmonic generation (HHG) in 20011,2, titanium doped sapphire (Ti:Sapphire) lasers centered at 800 nm have been the workhorse for attosecond research. Tese systems have enabled coherent pulses covering the extreme ultraviolet spectral range of 15–150 eV3,4. However, for many applications in ultrafast physics and chemistry it is instrumental to extend attosecond capabilities into and beyond the sof X-ray (SXR) “water window”, i.e., ≥530 eV. -
19 the Active Denial System (ADS) Is a Non-Lethal Weapons Technology
THE ACTIVE DENIAL SYSTEM: OBSTACLES AND PROMISE BENJAMIN BUCH AND KATHERINE MITCHELL The Active Denial System (ADS) is a non-lethal weapons technology that uses millimeter-wave directed energy to arrest and deter potential adversaries. Developed by the Air Force Research Laboratory and the Department of Defense’s Non-Lethal Weapons Program, ADS provides U.S. forces with a highly effective means of responding to potential threats while also preserving human life. Despite its promise, ADS has confronted non-technological challenges in its deployment, most recently in Afghanistan. This brief analyzes the political, psychological, and sociological barriers to the use of non-lethal directed energy weapons. Specifically, it surveys the psychological and sociological biases against radiation-based and non-lethal technology and how these prejudices were overcome in the past. It also examines potential human rights concerns and political complications that might arise from the deployment of ADS in population protection operations. Given these obstacles, the report proposes a series of recommendations for the use of ADS moving forward. The Changing Nature of Warfare The two decades following the conclusion of the Cold War have presented a new strategic and operational landscape for American military planners. The overwhelming conventional superiority of the United States has encouraged adversaries to adopt unconventional asymmetric strategies and tactics. Rise of Asymmetric Conflict Given U.S. conventional military supremacy, American forces have increasingly encountered adversaries who seek to exploit asymmetries in vulnerability, logistics, and organization through the novel use of strategy, tactics, and technology. This asymmetric form of warfare avoids traditional force-on-force confrontations in which U.S. -
Design of a Two-Stage Laser Pulse Shaping System for Fel Photoinjectors∗
Proceedings of FEL 2006, BESSY, Berlin, Germany THPPH026 DESIGN OF A TWO-STAGE LASER PULSE SHAPING SYSTEM FOR FEL PHOTOINJECTORS∗ M. B. Danailov, A. Demidovich, R. Ivanov (ELETTRA, Basovizza, Trieste). Abstract schemes clearer, we will very briefly introduce some This paper presents an approach for photoinjector laser basics of the pulse shaping theory. pulse shaping which combines the two main pulse shaping techniques, namely acousto-optic dispersive filter Assuming that the light field can be factorized into spatial (DAZZLER) and Fourier-based 4-f system. The and time dependent part, the electric field of the latter can DAZZLER is inserted between the seed mode-locked be written as: 1/2 oscillator and the amplifier and is used for preliminary E(t)=1/2 [I(t)] exp{i[ω0t-ϕ(t)]}+ c.c. , (1) shaping in the infrared, while the final pulse shape and duration are determined by a 4-f dispersive system 1/2 positioned after the harmonic conversion to UV. where ω0 is the central frequency , I(t) and ϕ (t) are the time dependent temporal amplitude and phase. For INTRODUCTION simplicity in the following equations the c.c. part will be Temporal pulse shaping is one of the most important omitted. The quantity 1/2 requirements to photoinjector lasers needed in the E(t)= I(t) exp[-iϕ(t)] (2) majority of FEL projects. The laser pulses commonly requested for excitation of the photocathode are in the UV is referred to as the complex amplitude in the time (around 260 nm) and have flat-top shape of duration in domain. -
China's Laser Weapons
www.rsis.edu.sg No. 093 – 5 June 2018 RSIS Commentary is a platform to provide timely and, where appropriate, policy-relevant commentary and analysis of topical and contemporary issues. The authors’ views are their own and do not represent the official position of the S. Rajaratnam School of International Studies, NTU. These commentaries may be reproduced with prior permission from RSIS and due recognition to the author(s) and RSIS. Please email to Mr Yang Razali Kassim, Editor RSIS Commentary at [email protected]. China’s Laser Weapons: Future Potential, Future Tensions? By Zi Yang Synopsis China’s increasingly sophisticated laser weapons arsenal is becoming a source of concern for its strategic rivals. What are their future potential? Commentary IN MAY 2018, the United States Department of Defence lodged a formal protest to China regarding a series of incidents in Djibouti where military-grade lasers were directed at US aircraft from China’s naval base, some 13 kilometres from Camp Lemonnier, the US’ only permanent base in East Africa. In one event, the pilot and co- pilot of a US Air Force C-130 Hercules were dazzled with lasers and sustained minor eye injuries. Spokespersons for China’s Ministry of National Defence and the Ministry of Foreign Affairs however have categorically denied US claims, labelling the concerns as “untrue criticisms”. Meanwhile, rhetoric has been heating-up in the public opinion sphere. US- based authors have criticised China’s insincerity in working to resolve the lasing issue, while Chinese writers shot back at “false accusations” or outright celebrated the triumph of Chinese laser weapons over “arrogant Americans” spying on China’s naval base.