I a STUDY of the MECHANISM by WHICH CD86 REGULATES Igg1
Total Page:16
File Type:pdf, Size:1020Kb
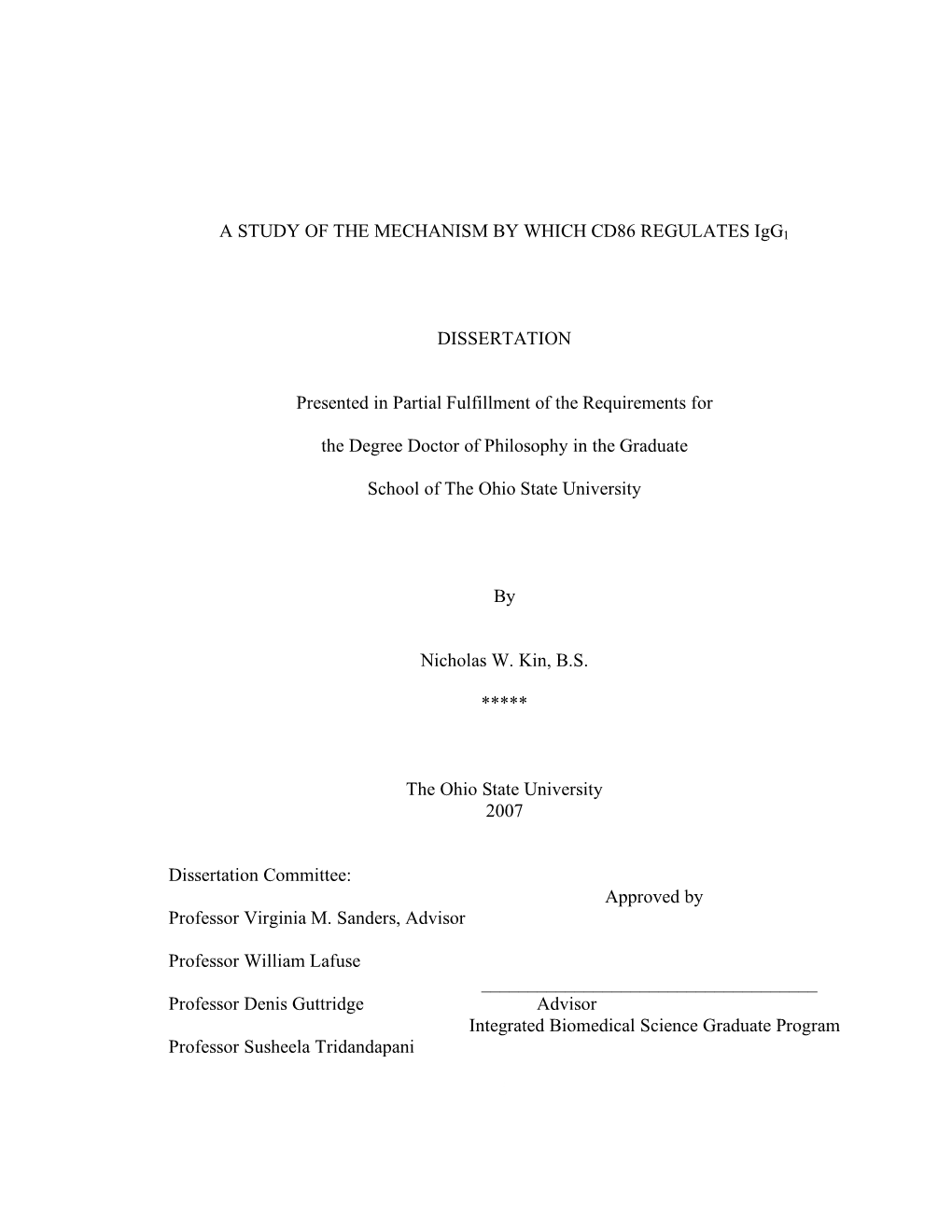
Load more
Recommended publications
-
Immunomodulation and Generation of Tolerogenic Dendritic Cells by Probiotic Bacteria in Patients with Inflammatory Bowel Disease
International Journal of Molecular Sciences Article Immunomodulation and Generation of Tolerogenic Dendritic Cells by Probiotic Bacteria in Patients with Inflammatory Bowel Disease 1, 2, 1 Shaghayegh Baradaran Ghavami y, Abbas Yadegar y , Hamid Asadzadeh Aghdaei , Dario Sorrentino 3,4,*, Maryam Farmani 1 , Adil Shamim Mir 5, Masoumeh Azimirad 2 , Hedieh Balaii 6, Shabnam Shahrokh 6 and Mohammad Reza Zali 6 1 Basic and Molecular Epidemiology of Gastrointestinal Disorders Research Center, Research Institute for Gastroenterology and Liver Diseases, Shahid Beheshti University of Medical Sciences, Tehran 1985717413, Iran; [email protected] (S.B.G.); [email protected] (H.A.A.); [email protected] (M.F.) 2 Foodborne and Waterborne Diseases Research Center, Research Institute for Gastroenterology and Liver Diseases, Shahid Beheshti University of Medical Sciences, Tehran 1985717413, Iran; [email protected] (A.Y.); [email protected] (M.A.) 3 IBD Center, Division of Gastroenterology, Virginia Tech Carilion School of Medicine, Roanoke, VA 24016, USA 4 Department of Clinical and Experimental Medical Sciences, University of Udine School of Medicine, 33100 Udine, Italy 5 Department of Internal Medicine, Roanoke Memorial Hospital, Carilion Clinic, VA 24014, USA; [email protected] 6 Gastroenterology and Liver Diseases Research Center, Research Institute for Gastroenterology and Liver Diseases, Shahid Beheshti University of Medical Sciences, Tehran 1985717413, Iran; [email protected] (H.B.); [email protected] (S.S.); [email protected] (M.R.Z.) * Correspondence: [email protected] These authors equally contributed to this study. y Received: 7 August 2020; Accepted: 27 August 2020; Published: 29 August 2020 Abstract: In inflammatory bowel diseases (IBD), the therapeutic benefit and mucosal healing from specific probiotics may relate to the modulation of dendritic cells (DCs). -
B Cell Checkpoints in Autoimmune Rheumatic Diseases
REVIEWS B cell checkpoints in autoimmune rheumatic diseases Samuel J. S. Rubin1,2,3, Michelle S. Bloom1,2,3 and William H. Robinson1,2,3* Abstract | B cells have important functions in the pathogenesis of autoimmune diseases, including autoimmune rheumatic diseases. In addition to producing autoantibodies, B cells contribute to autoimmunity by serving as professional antigen- presenting cells (APCs), producing cytokines, and through additional mechanisms. B cell activation and effector functions are regulated by immune checkpoints, including both activating and inhibitory checkpoint receptors that contribute to the regulation of B cell tolerance, activation, antigen presentation, T cell help, class switching, antibody production and cytokine production. The various activating checkpoint receptors include B cell activating receptors that engage with cognate receptors on T cells or other cells, as well as Toll-like receptors that can provide dual stimulation to B cells via co- engagement with the B cell receptor. Furthermore, various inhibitory checkpoint receptors, including B cell inhibitory receptors, have important functions in regulating B cell development, activation and effector functions. Therapeutically targeting B cell checkpoints represents a promising strategy for the treatment of a variety of autoimmune rheumatic diseases. Antibody- dependent B cells are multifunctional lymphocytes that contribute that serve as precursors to and thereby give rise to acti- cell- mediated cytotoxicity to the pathogenesis of autoimmune diseases -
Expression in B Cells Receptor-Induced Regulation of B7-2
The Journal of Immunology  B Cell Receptor- and 2-Adrenergic Receptor-Induced Regulation of B7-2 (CD86) Expression in B Cells1 Adam P. Kohm,2* Afsaneh Mozaffarian,3* and Virginia M. Sanders4*† The costimulatory molecule B7-2 (CD86) is expressed on the surface of APCs, including B cells. Considering the importance of B7-2 in regulating both T and B cell function, it may be important to understand the regulatory mechanisms governing its  expression. We report in this study that stimulation of the B cell receptor (BCR) and/or a neurotransmitter receptor, the 2-   adrenergic receptor ( 2AR), may cooperate to regulate B cell-associated B7-2 expression in vitro and in vivo. 2AR stimulation further enhanced the level of BCR-induced B7-2 expression in B cells potentially via protein tyrosine kinase-, protein kinase A-,  protein kinase C-, and mitogen-activated protein kinase-dependent mechanisms. Importantly, BCR and/or 2AR stimulation, but not histone hyperacetylation and DNA hypomethylation alone, increased B cell-associated B7-2 expression by increasing B7-2 mRNA stability, NF-B nuclear binding, and NF-B-dependent gene transcription. Thus, this study provides additional insight  into the signaling intermediates and molecular mechanisms by which stimulation of the BCR and 2AR may regulate B cell- associated B7-2 expression. The Journal of Immunology, 2002, 168: 6314–6322. he growing B7 family of costimulatory molecules criti- tion increased the level of B7-2 mRNA and protein expression in cally influences the T cell-dependent Ab response. The the B cell with peak expression at 12 and 24 h, respectively (5–9). -
Induces Antigen Presentation in B Cells Cell-Activating Factor of The
B Cell Maturation Antigen, the Receptor for a Proliferation-Inducing Ligand and B Cell-Activating Factor of the TNF Family, Induces Antigen Presentation in B Cells This information is current as of September 27, 2021. Min Yang, Hidenori Hase, Diana Legarda-Addison, Leena Varughese, Brian Seed and Adrian T. Ting J Immunol 2005; 175:2814-2824; ; doi: 10.4049/jimmunol.175.5.2814 http://www.jimmunol.org/content/175/5/2814 Downloaded from References This article cites 54 articles, 36 of which you can access for free at: http://www.jimmunol.org/content/175/5/2814.full#ref-list-1 http://www.jimmunol.org/ Why The JI? Submit online. • Rapid Reviews! 30 days* from submission to initial decision • No Triage! Every submission reviewed by practicing scientists • Fast Publication! 4 weeks from acceptance to publication by guest on September 27, 2021 *average Subscription Information about subscribing to The Journal of Immunology is online at: http://jimmunol.org/subscription Permissions Submit copyright permission requests at: http://www.aai.org/About/Publications/JI/copyright.html Email Alerts Receive free email-alerts when new articles cite this article. Sign up at: http://jimmunol.org/alerts The Journal of Immunology is published twice each month by The American Association of Immunologists, Inc., 1451 Rockville Pike, Suite 650, Rockville, MD 20852 Copyright © 2005 by The American Association of Immunologists All rights reserved. Print ISSN: 0022-1767 Online ISSN: 1550-6606. The Journal of Immunology B Cell Maturation Antigen, the Receptor for a Proliferation-Inducing Ligand and B Cell-Activating Factor of the TNF Family, Induces Antigen Presentation in B Cells1 Min Yang,* Hidenori Hase,* Diana Legarda-Addison,* Leena Varughese,* Brian Seed,† and Adrian T. -
A Distinct Subset of Plasmacytoid Dendritic Cells Induces Activation and Differentiation of B and T Lymphocytes
A distinct subset of plasmacytoid dendritic cells induces activation and differentiation of B and T lymphocytes Hong Zhanga, Josh D. Gregoriob, Toru Iwahoric, Xiangyue Zhanga, Okmi Choid, Lorna L. Tolentinod, Tyler Prestwooda, Yaron Carmia, and Edgar G. Englemana,1 aDepartment of Pathology, Stanford University School of Medicine, Stanford, CA 94304; bKyowa Kirin Pharmaceutical Research, La Jolla, CA 92037; cSoulage Medical Clinic, Inage-Ku Chiba-City, Chiba, 263-0005, Japan; and dStanford Blood Center, Stanford Hospital, Stanford, CA 94304 Edited by Kenneth M. Murphy, Washington University, St. Louis, MO, and approved January 10, 2017 (received for review June 29, 2016) Plasmacytoid dendritic cells (pDCs) are known mainly for their not only in peripheral blood mononuclear cells (PBMCs), but secretion of type I IFN upon viral encounter. We describe a also in bone marrow, cord blood, and tonsil, and can be gener- + + + CD2hiCD5 CD81 pDC subset, distinguished by prominent den- ated from CD34 hematopoietic progenitor cells in vitro. drites and a mature phenotype, in human blood, bone marrow, and tonsil, which can be generated from CD34+ progenitors. These Results + + CD2hiCD5 CD81 cells express classical pDC markers, as well as the CD5 and CD81 Distinguish Subsets of Human pDCs. Peripheral blood toll-like receptors that enable conventional pDCs to respond to pDCs were analyzed by flow cytometry for their expression of viral infection. However, their gene expression profile is distinct, different tetraspanin proteins based on the previous finding that and they produce little or no type I IFN upon stimulation with expression of the tetraspanin molecule CD9 distinguishes high CpG oligonucleotides, likely due to their diminished expression of and low IFNα-producing pDCs in mice (23). -
Innate and Adaptive Signals Enhance Differentiation and Expansion of Dual-Antibody Autoreactive B Cells in Lupus
ARTICLE DOI: 10.1038/s41467-018-06293-z OPEN Innate and adaptive signals enhance differentiation and expansion of dual-antibody autoreactive B cells in lupus Allison Sang1, Thomas Danhorn 2, Jacob N. Peterson1, Andrew L. Rankin3,4, Brian P. O’Connor1,2,5,6, Sonia M. Leach2,5, Raul M. Torres1,5 & Roberta Pelanda1,5 1234567890():,; Autoreactive B cells have a major function in autoimmunity. A small subset of B cells expressing two distinct B-cell-antigen-receptors (B2R cells) is elevated in many patients with systematic lupus erythematosus (SLE) and in the MRL(/lpr) mouse model of lupus, and is often autoreactive. Here we show, using RNAseq and in vitro and in vivo analyses, signals that are required for promoting B2R cell numbers and effector function in autoimmune mice. Compared with conventional B cells, B2R cells are more responsive to Toll-like receptor 7/9 and type I/II interferon treatment, display higher levels of MHCII and co-receptors, and depend on IL-21 for their homeostasis; moreover they expand better upon T cell-dependent antigen stimulation, and mount a more robust memory response, which are characteristics essential for enhanced (auto)immune responses. Our findings thus provide insights on the stimuli for the expansion of an autoreactive B cell subset that may contribute to the etiology of SLE. 1 Department of Immunology and Microbiology, University of Colorado School of Medicine, Aurora, CO 80045, USA. 2 Center for Genes, Environment and Health, National Jewish Health, Denver, CO 80206, USA. 3 Inflammation and Immunology, Pfizer Research, Cambridge, MA 02140, USA. 4 Immuno- Oncology Discovery, FivePrime Therapeutics, South San Francisco, CA 94080, USA. -
Activation of Tumor-Tolerized CD8 T Cells CD80/CD86 in Dendritic Cell
Published July 13, 2012, doi:10.4049/jimmunol.1201271 The Journal of Immunology Differential Requirement for CD70 and CD80/CD86 in Dendritic Cell-Mediated Activation of Tumor-Tolerized CD8 T Cells S. Peter Bak,*,† Mike Stein Barnkob,* Ailin Bai,*,†,1 Eileen M. Higham,*,‡,2 K. Dane Wittrup,*,‡,x and Jianzhu Chen*,† A major obstacle to efficacious T cell-based cancer immunotherapy is the tolerizing-tumor microenvironment that rapidly inacti- vates tumor-infiltrating lymphocytes. In an autochthonous model of prostate cancer, we have previously shown that intratumoral injection of Ag-loaded dendritic cells (DCs) delays T cell tolerance induction as well as refunctionalizes already tolerized T cells in the tumor tissue. In this study, we have defined molecular interactions that mediate the effects of DCs. We show that pretreating Ag- loaded DCs with anti-CD70 Ab abolishes the ability of DCs to delay tumor-mediated T cell tolerance induction, whereas interfering with 4-1BBL, CD80, CD86, or both CD80 and CD86 had no significant effect. In contrast, CD802/2 or CD802/2CD862/2 DCs failed to reactivate already tolerized T cells in the tumor tissue, whereas interfering with CD70 and 4-1BBL had no effect. Furthermore, despite a high level of programmed death 1 expression by tumor-infiltrating T cells and programmed death ligand 1 expression in the prostate, disrupting programmed death 1/programmed death ligand 1 interaction did not enhance T cell function in this model. These findings reveal dynamic requirements for costimulatory signals to overcome tumor-induced tolerance and have significant implications for developing more effective cancer immunotherapies. The Journal of Immunology, 2012, 189: 000–000. -
Tetraspanin CD81 Promotes Tumor Growth and Metastasis by Modulating the Functions of T Regulatory and Myeloid-Derived Suppressor Cells
Author Manuscript Published OnlineFirst on September 1, 2015; DOI: 10.1158/0008-5472.CAN-15-1021 Author manuscripts have been peer reviewed and accepted for publication but have not yet been edited. Tetraspanin CD81 promotes tumor growth and metastasis by modulating the functions of T regulatory and myeloid-derived suppressor cells Felipe Vences-Catalán1, Ranjani Rajapaksa1, Minu K. Srivastava1, Aurelien Marabelle1*, Chiung-Chi Kuo1, Ronald Levy1 and Shoshana Levy1 1Department of Medicine, Division of Oncology, Stanford University Medical Center, Stanford, CA, USA Current addresses: * Gustave Roussy Cancer Campus, Villejuif, France; INSERM U1015, Villejuif, France Short title: CD81 promotes tumor growth and metastasis Keywords: Tetraspanin; CD81; Metastasis; Tregs; MDSC; immune suppression GRANT SUPPORT This work was supported by the Translational Cancer Award from Stanford Cancer Institute and the Breast Cancer Research program from the Department of Defense grant W81XWH-14-1-0397 (both grants were awarded to S. Levy). None of the authors have a conflict of interest. Corresponding Author Shoshana Levy Department of Medicine, Division of Oncology, Stanford University Medical Center, Stanford, CA, 94305, USA [email protected] Phone: 650-725-6425 Fax: 650- 736-1454 Downloaded from cancerres.aacrjournals.org on September 28, 2021. © 2015 American Association for Cancer Research. Author Manuscript Published OnlineFirst on September 1, 2015; DOI: 10.1158/0008-5472.CAN-15-1021 Author manuscripts have been peer reviewed and accepted for publication but have not yet been edited. CD81 promotes tumor growth and metastasis SUMMARY Tumor cells counteract innate and adaptive antitumor immune responses by recruiting regulatory T cells (Treg) and innate myeloid-derived suppressor cells (MDSC), which facilitate immune escape and metastatic dissemination. -
Transcriptional Regulation of CD40 Expression by 4 Ribosomal Proteins Via a Functional SNP on a Disease-Associated CD40 Locus
G C A T T A C G G C A T genes Article Transcriptional Regulation of CD40 Expression by 4 Ribosomal Proteins via a Functional SNP on a Disease-Associated CD40 Locus 1,2, 1, 1 1,3 1,4 1 Meijuan Zou z, Xiaoyu Zhang z, Danli Jiang , Yihan Zhao , Ting Wu , Qiaoke Gong , 5 6 7, 1,8, Hang Su , Di Wu , Larry Moreland y and Gang Li * 1 Aging Institute at University of Pittsburgh Medical Center, Pittsburgh, PA 15219, USA; [email protected] (M.Z.); [email protected] (X.Z.); [email protected] (D.J.); [email protected] (Y.Z.); [email protected] (T.W.); [email protected] (Q.G.) 2 Department of Pharmacology, Nanjing Medical University, Nanjing 211166, China 3 School of Life Science, East China Normal University, Shanghai 200062, China 4 Department of Medicine, Xiangya School of Medicine, Central South University, Changsha 410083, China 5 Bioinformatics and Computational Biology, University of North Carolina, Chapel Hill, NC 27514, USA; [email protected] 6 Department of Periodontology, University of North Carolina at Chapel Hill, Chapel Hill, NC 27599, USA; [email protected] 7 Division of Rheumatology, Department of Medicine, University of Pittsburgh, Pittsburgh, PA 15219, USA; [email protected] 8 Division of Cardiology, Department of Medicine, University of Pittsburgh, Pittsburgh, PA 15219, USA * Correspondence: [email protected]; Tel.: +01-857-472-4787 Current address: Department of Medicine, Division of Rheumatology, University of Colorado, y Aurora, CO 80045, USA. These authors contributed equally to this work. z Received: 26 October 2020; Accepted: 14 December 2020; Published: 21 December 2020 Abstract: Previously, using FREP-MS, we identified a protein complex including eight proteins that specifically bind to the functional SNP (fSNP) rs6032664 at a CD40 locus associated with autoimmune diseases. -
Functional Characterization of Potential New Agents for Cancer Immunotherapy
Functional Characterization of Potential New Agents for Cancer Immunotherapy The Harvard community has made this article openly available. Please share how this access benefits you. Your story matters Citable link http://nrs.harvard.edu/urn-3:HUL.InstRepos:39010116 Terms of Use This article was downloaded from Harvard University’s DASH repository, and is made available under the terms and conditions applicable to Other Posted Material, as set forth at http:// nrs.harvard.edu/urn-3:HUL.InstRepos:dash.current.terms-of- use#LAA Functional Characterization of Potential New Agents for Cancer Immunotherapy Xiaoxu Wang A Thesis Submitted to the Faculty of The Harvard Medical School in Partial Fulfillment of the Requirements for the Degree of Master of Medical Sciences in Immunology Harvard University Boston, Massachusetts. May, 2018 Thesis Advisor: Dr. Gordon Freeman Xiaoxu Wang Functional Characterization of Potential New Agents for Cancer Immunotherapy Abstract PD-1/PD-L1 checkpoint blockade has demonstrated promise in a variety of malignancies. The blockade of T cell inhibitory molecules PD-1 and PD-L1 can rejuvenate T cells and improve tumor cell killing. However, the overall response rate of PD-1/PD-L1 immunotherapy is only about 20-30%. One promising method to improve cancer immunotherapy is combinatory immunotherapies that target new receptors/ligands regulating T cell activity, so the functional characterization of these new receptors/ligands is urgent. Due to the functional importance of the B7 superfamily in T cell activation, I studied T cell regulating functions of two poorly characterized B7 superfamily members, IgC domain-truncated CD86 and butyrophilin-like 2 (BTNL2). -
And Macrophage-CSF − Defining GM-CSF
Defining GM-CSF− and Macrophage-CSF− Dependent Macrophage Responses by In Vitro Models This information is current as Derek C. Lacey, Adrian Achuthan, Andrew J. Fleetwood, of October 1, 2021. Hang Dinh, John Roiniotis, Glen M. Scholz, Melody W. Chang, Sandra K. Beckman, Andrew D. Cook and John A. Hamilton J Immunol 2012; 188:5752-5765; Prepublished online 30 April 2012; doi: 10.4049/jimmunol.1103426 Downloaded from http://www.jimmunol.org/content/188/11/5752 Supplementary http://www.jimmunol.org/content/suppl/2012/04/30/jimmunol.110342 http://www.jimmunol.org/ Material 6.DC1 References This article cites 50 articles, 20 of which you can access for free at: http://www.jimmunol.org/content/188/11/5752.full#ref-list-1 Why The JI? Submit online. by guest on October 1, 2021 • Rapid Reviews! 30 days* from submission to initial decision • No Triage! Every submission reviewed by practicing scientists • Fast Publication! 4 weeks from acceptance to publication *average Subscription Information about subscribing to The Journal of Immunology is online at: http://jimmunol.org/subscription Permissions Submit copyright permission requests at: http://www.aai.org/About/Publications/JI/copyright.html Email Alerts Receive free email-alerts when new articles cite this article. Sign up at: http://jimmunol.org/alerts The Journal of Immunology is published twice each month by The American Association of Immunologists, Inc., 1451 Rockville Pike, Suite 650, Rockville, MD 20852 Copyright © 2012 by The American Association of Immunologists, Inc. All rights reserved. Print ISSN: 0022-1767 Online ISSN: 1550-6606. The Journal of Immunology Defining GM-CSF– and Macrophage-CSF–Dependent Macrophage Responses by In Vitro Models Derek C. -
Intrarenal Antigens Activate CD4 Cells Via Co-Stimulatory
JASN Express. Published on January 9, 2008 as doi: 10.1681/ASN.2007030386 BASIC RESEARCH www.jasn.org Intrarenal Antigens Activate CD4؉ Cells via Co-stimulatory Signals from Dendritic Cells Kristy L. Edgtton,* Joshua Y. Kausman,* Ming Li,* Kim O’Sullivan,* Cecilia Lo,* Paul Hutchinson,* Hideo Yagita,† Stephen R. Holdsworth,* and A. Richard Kitching* *Centre for Inflammatory Diseases, Monash University Department of Medicine, Monash Medical Centre, Clayton, Victoria, Australia; and †Department of Immunology, Juntendo University School of Medicine, Tokyo, Japan ABSTRACT Dendritic cells in the kidney take up antigens, but little is known about their role in providing co- ϩ stimulatory signals for the activation of CD4 cells. This study examined the phenotype of dendritic cells in the renal interstitium and in the lymph node draining the kidney before and after intrarenal ovalbumin injection. After intrarenal injection of the antigen, expression of the co-stimulatory molecules CD86 and programmed cell death ligand 1 (PD-L1) increased on renal dendritic cells, whereas expression of only CD86 increased on dendritic cells of the draining lymph node. The activation and proliferation of ϩ antigen-specific CD4 cells in the lymph node were assessed by transfer of naı¨ve,fluorescently labeled ovalbumin-specific T cell receptor transgenic cells to mice before antigen administration. Blocking both ϩ CD86 and CD80 profoundly inhibited CD4 cell proliferation, but CD86 was the dominant CD28 ligand ϩ in the early proliferative response of CD4 cells. Conversely, activation of PD-1, the receptor expressed ϩ ϩ on CD4 cells that binds PD-L1 and PD-L2, reduced the proliferation of CD4 cells in the draining lymph ϩ node.