The Proton-Electron Mass Ratio, the Fine-Structure Constant, the Electron G-Factor, and Von Klitzing’S Constant
Total Page:16
File Type:pdf, Size:1020Kb
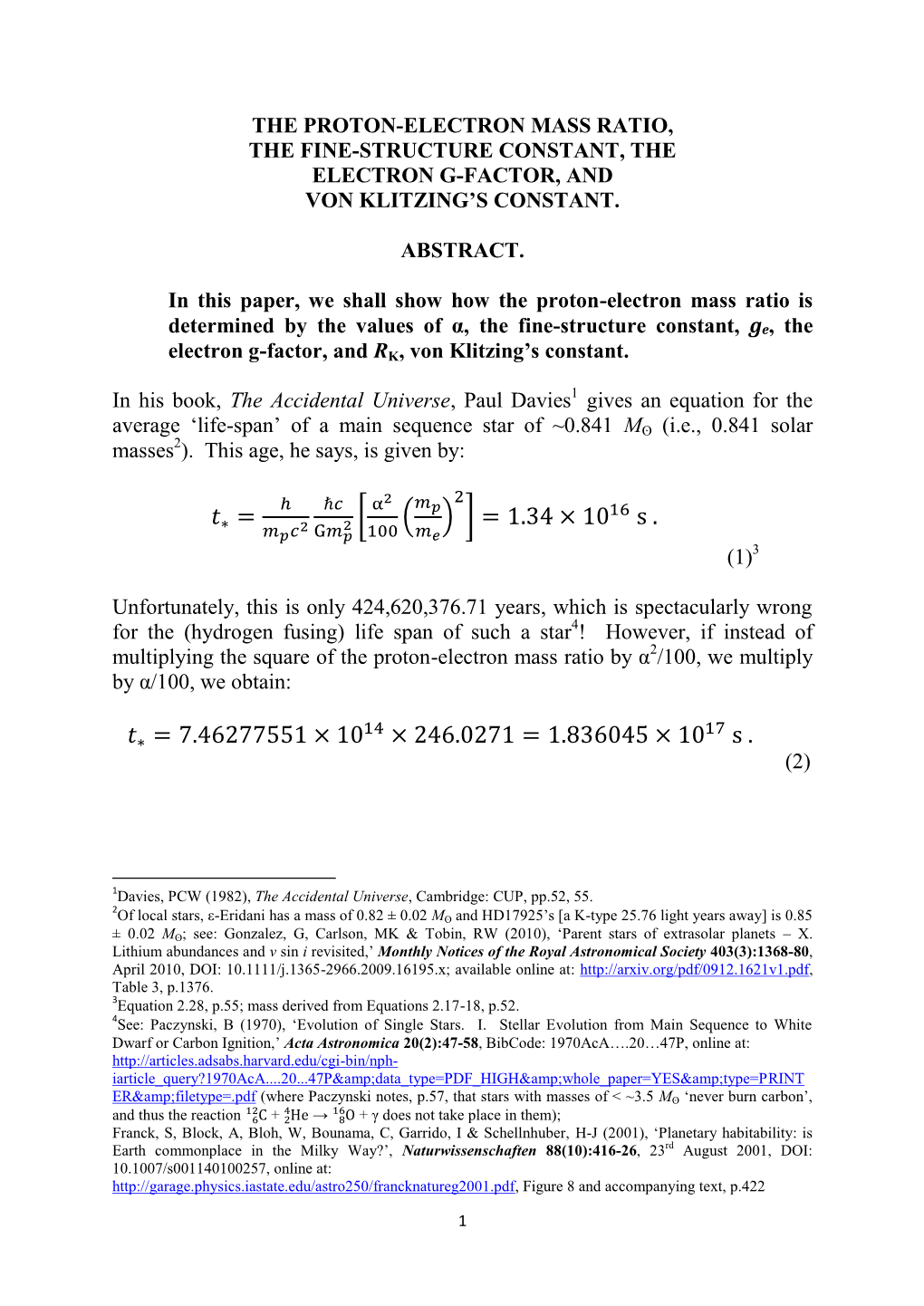
Load more
Recommended publications
-
Anthropic Explanations in Cosmology
View metadata, citation and similar papers at core.ac.uk brought to you by CORE provided by PhilSci Archive 1 Jesús Mosterín ANTHROPIC EXPLANATIONS IN COSMOLOGY In the last century cosmology has ceased to be a dormant branch of speculative philosophy and has become a vibrant part of physics, constantly invigorated by new empirical inputs from a legion of new terrestrial and outer space detectors. Nevertheless cosmology continues to be relevant to our philosophical world view, and some conceptual and methodological issues arising in cosmology are in need of epistemological analysis. In particular, in the last decades extraordinary claims have been repeatedly voiced for an alleged new type of scientific reasoning and explanation, based on a so-called “anthropic principle”. “The anthropic principle is a remarkable device. It eschews the normal methods of science as they have been practiced for centuries, and instead elevates humanity's existence to the status of a principle of understanding” [Greenstein 1988, p. 47]. Steven Weinberg has taken it seriously at some stage, while many physicists and philosophers of science dismiss it out of hand. The whole issue deserves a detailed critical analysis. Let us begin with a historical survey. History of the anthropic principle After Herman Weyl’s remark of 1919 on the dimensionless numbers in physics, several eminent British physicists engaged in numerological or aprioristic speculations in the 1920's and 1930's (see Barrow 1990). Arthur Eddington (1923) calculated the number of protons and electrons in the universe (Eddington's number, N) and found it to be around 10 79 . He noticed the coincidence between N 1/2 and the ratio of the electromagnetic to gravitational 2 1/2 39 forces between a proton and an electron: e /Gm emp ≈ N ≈ 10 . -
Remarkable Properties of the Eddington Number 137 and Electric Parameter 137.036 Excluding the Multiverse Hypothesis Francis M
Remarkable Properties of the Eddington Number 137 and Electric Parameter 137.036 excluding the Multiverse Hypothesis Francis M. Sanchez February-May 2015 Abstract. Considering that the Large Eddington Number has correctly predicted the number of atoms in the Universe, the properties of the Eddington electric number 137 are studied. This number shows abnormal arithmetic properties, in liaison with the 5th harmonic number 137/60. It seems that Egyptians was aware of this, as the architecture of the Hypostyle Karnak room reveals, as well as the Ptolemaic approximation π ≈ 2 + 137/120, together with a specific mention in the Bible, and overhelming connexions with musical canonic numbers. The SO(32) characteristic superstring number 496, the third perfect number, connects directly with the three main interaction parameters, and is very close to the square root of the Higgs boson-electron mass ratio, so the Higgs boson discovery excludes the Multiverse, favoring rather a unique Cosmos being a finite computer using 137 and its extension 137.036 as calculation basis. The direct liaison between the mean value of the two main cosmic radiuses and the Bohr radius through the simplest harmonic series excludes any role of chance. Precise symmetric relations involving the Kotov non-Doppler period permit to propose precise (100 ppb) values for the weak and strong interaction constants, as well as G ≈ 6.675464346 × 10-11 kg-1 m3 s-2, at 2σ higher than the tabulated value.. This value is confirmed by a direct connection with the Superspeed ratio C/c ≈ 6.945480 × 1060. 1. Eddington and 137 Eddington has established [1] that, in reduced units, the square of the inverse electric charge must be 137. -
Remarkables Properties of the Eddington Number 137 and Electric Parameter 137.036 Excluding the Multiverse Hypothesis Francis M
Remarkables Properties of the Eddington Number 137 and Electric Parameter 137.036 excluding the Multiverse Hypothesis Francis M. Sanchez, February 2015 Abstract. Considering that the Large Eddington Number has correctly predicted the number of atoms in the Universe, the properties of the Eddington electric number 137 are studied. This number shows abnormal arithmetic properties, in liaison with canonic numbers of the string and superstring theories including overwhelming relations with musical canonic numbers. The liaison with the harmonic series is so tight that it seems that Egyptians was aware of them, as the architecture of the Hypostyle Karnak room reveals. The direct liaison between the mean value of two Universe radiuses and the Bohr radius through the simplest harmonic series excludes any role of chance. It is concluded that the Cosmos is a finite computer using 137 and its extension 137.036 as calculation basis. 1. The Large Eddington Number: the most astonishing prediction in Physics of all times Eddington believed he had identified an algebraic basis for fundamental physics, which he termed "E-numbers" (representing a certain group– a Clifford algebra). These in effect incorporated spacetime into a higher-dimensional structure. While his theory has long been neglected by the general physics community, similar algebraic notions underlie many modern attempts at a grand unified theory. Moreover, Eddington's emphasis on the values of the fundamental constants, and specifically upon dimensionless numbers derived from them, is nowadays a central concern of physics. In particular, he predicted a number of hydrogen atoms in the Universe [1] 136 x 2256, or equivalently the half of the total number of particles protons + electrons. -
(Owen Willans) Richardson
O. W. (Owen Willans) Richardson: An Inventory of His Papers at the Harry Ransom Center Descriptive Summary Creator: Richardson, O. W. (Owen Willans), 1879-1959 Title: O. W. (Owen Willans) Richardson Papers Dates: 1898-1958 (bulk 1920-1940) Extent: 112 document boxes, 2 oversize boxes (49.04 linear feet), 1 oversize folder (osf), 5 galley folders (gf) Abstract: The papers of Sir O. W. (Owen Willans) Richardson, the Nobel Prize-winning British physicist who pioneered the field of thermionics, contain research materials and drafts of his writings, correspondence, as well as letters and writings from numerous distinguished fellow scientists. Call Number: MS-3522 Language: Primarily English; some works and correspondence written in French, German, or Italian . Note: The Ransom Center gratefully acknowledges the assistance of the Center for History of Physics, American Institute of Physics, which provided funds to support the processing and cataloging of this collection. Access: Open for research Administrative Information Additional The Richardson Papers were microfilmed and are available on 76 Physical Format reels. Each item has a unique identifying number (W-xxxx, L-xxxx, Available: R-xxxx, or M-xxxx) that corresponds to the microfilm. This number was recorded on the file folders housing the papers and can also be found on catalog slips present with each item. Acquisition: Purchase, 1961 (R43, R44) and Gift, 2005 Processed by: Tessa Klink and Joan Sibley, 2014 Repository: The University of Texas at Austin, Harry Ransom Center Richardson, O. W. (Owen Willans), 1879-1959 MS-3522 2 Richardson, O. W. (Owen Willans), 1879-1959 MS-3522 Biographical Sketch The English physicist Owen Willans Richardson, who pioneered the field of thermionics, was also known for his work on photoelectricity, spectroscopy, ultraviolet and X-ray radiation, the electron theory, and quantum theory. -
A Bio-Info-Digital Universe Model (BIDUM
1 A Bio-Info-Digital Universe Model (BIDUM, version 1.0) inspired by a Teller’s large number hypothesis overlooked by the great majority of physicists (including Tipler, Barrow, Dirac and Einstein) but also Teller himself * (Open development interval: 2008 – 2015 - ?) * Andrei Lucian Dr ăgoi 1,2,3 * Motto: "[God]: universe is nothing but a big copying machine, reproducing your thoughts [pure information] in physical form [energy/matter], that will be your experience” 4 (Neale Donald Walsch, Conversations with God, 3rd volume) Abstract „Edward Teller appears to have been the first who speculate that there may exist a logarithmic 2 -39 relation between the fine structure constant (α) and the parameter G ·mN /h ·c~10 of the form 2 -1 60 α~ln(G ·mN /h ·c) (in fact α =ln(3.17 x 10 and the formula is too insensitive to be of very much use in predicting exact relations)“ 5. I will try to demonstrate that Barrow and Tipler (but also the great majority of the physicists including those mentioned in the title) overlooked the possibility that Teller’s hypothesis (called “speculation”) may be much more inspired and profound than the Dirac’s large number hypothesis (DLNH) , as it can offer an elegant explanation both to the fine structure constant (FSC) and to DLNH (using FSC) and also can predict a Planck-like gravitational constants series and a big G series (a G “imprint” for every type of atom in any state) as a unified quantum-relativistic theory of quantum gravity that is experimentally testable. IDUM proposes an informational view on the universe in which all physical constants can be derived (at least theoretically) from just one parameter: the total information of the universe (a quantum fluctuation resulted from a huge informational input to a “nothing” 0D vacuum). -
Arxiv:1411.4673V1 [Hep-Ph] 17 Nov 2014 E Unn Coupling Running QED Scru S Considerable and Challenge
Attempts at a determination of the fine-structure constant from first principles: A brief historical overview U. D. Jentschura1, 2 and I. N´andori2 1Department of Physics, Missouri University of Science and Technology, Rolla, Missouri 65409-0640, USA 2MTA–DE Particle Physics Research Group, P.O.Box 51, H–4001 Debrecen, Hungary It has been a notably elusive task to find a remotely sensical ansatz for a calculation of Sommer- feld’s electrodynamic fine-structure constant αQED ≈ 1/137.036 based on first principles. However, this has not prevented a number of researchers to invest considerable effort into the problem, despite the formidable challenges, and a number of attempts have been recorded in the literature. Here, we review a possible approach based on the quantum electrodynamic (QED) β function, and on algebraic identities relating αQED to invariant properties of “internal” symmetry groups, as well as attempts to relate the strength of the electromagnetic interaction to the natural cutoff scale for other gauge theories. Conjectures based on both classical as well as quantum-field theoretical considerations are discussed. We point out apparent strengths and weaknesses of the most promi- nent attempts that were recorded in the literature. This includes possible connections to scaling properties of the Einstein–Maxwell Lagrangian which describes gravitational and electromagnetic interactions on curved space-times. Alternative approaches inspired by string theory are also dis- cussed. A conceivable variation of the fine-structure constant with time would suggest a connection of αQED to global structures of the Universe, which in turn are largely determined by gravitational interactions. PACS: 12.20.Ds (Quantum electrodynamics — specific calculations) ; 11.25.Tq (Gauge field theories) ; 11.15.Bt (General properties of perturbation theory) ; 04.60.Cf (Gravitational aspects of string theory) ; 06.20.Jr (Determination of fundamental constants) . -
Coupling the Permeability and Permittivity of Space to the Electron Orbital Time: Potential Phase Shift Between Entanglement Latency and the Universe’S Final Epoch
International Journal of Astrophysics and Space Science 2017; 5(1): 1-5 http://www.sciencepublishinggroup.com/j/ijass doi: 10.11648/j.ijass.20170501.11 ISSN: 2376-7014 (Print); ISSN: 2376-7022 (Online) Coupling the Permeability and Permittivity of Space to the Electron Orbital Time: Potential Phase Shift Between Entanglement Latency and the Universe’s Final Epoch Michael Persinger *, Trevor Carniello Biophysics Laboratory, Biomolecular Sciences Program, Laurentian University, Sudbury, Canada Email address: [email protected] (M. Persinger) *Corresponding author To cite this article: Michael Persinger, Trevor Carniello. Coupling the Permeability and Permittivity of Space to the Electron Orbital Time: Potential Phase Shift Between Entanglement Latency and the Universe’s Final Epoch. International Journal of Astrophysics and Space Science. Vol. 5, No. 1, 2017, pp. 1-5. doi: 10.11648/j.ijass.20170501.11 Received : November 30, 2016; Accepted : December 10, 2016; Published : February 13, 2017 Abstract: The strength of the magnetic field for different ratios of matter densities relative to the permittivity of a vacuum solves for values approaching the velocity of light. When the strength of the field associated with densities similar to liquid water, ice, or stars (such as the Sun) is considered with respect to the magnetic permeability and average mass density of the universe, the emergent velocity is ~10 23 m·s -1. This value has been derived from several approaches as the latency for excess correlation or “entanglement” and is consistent with a process that might explain the integrity of large-scale spatial structure over distances that are within fractions of the universe’s present diameter. -
The In-Formation Field Hypothesis
Electronic Journal of Sociology (2007) ISSN: 1198 3655 The In-formation Field Hypothesis Ervin Laszlo* The General Evolution Research Group Abstract There appears to be an as yet unexplained form and level in coherence in the various domains of nature. This anomalous phenomenon involves a quasi-instant transmission of an active kind of information that correlates entities across space and over time. The first part of this paper presents evidence that this nonlocal form of coherence is widespread in nature, occurring in the microdomain of the quantum, in the macrodomain of the universe, as well as in the mesodomains of life and mind. The second part argues (i) that the nonlocal coherence phenomenon is logically interpreted as the transmission of “in-formation,” a non-vectorial yet physically effective form of information, and (ii) that this transmission is realistically viewed as the effect of a universal in-formation field in nature. The Principal Proposition This paper presents evidence that phenomena obtain in the principal domains of observation that cannot be adequately explained in reference to presently recognized fields. The phenomena consist of a form of coherence that presupposes the transmission of information beyond the known physical or physico-chemical channels. The pertinent form of coherence suggests that the elements of the coherent system are nonlocally connected, i.e., are mutually “entangled.” This * Villa Franatoni 56040 Montescudaio (Prov. Pisa) Italy <[email protected]> implies, in turn, the presence of a particular form of information termed “in- formation” in nature. In a realistic interpretation the presence of in-formation in the various domains of observation provides warrant for postulating an additional universal field: the universal in-formation field. -
Comparison of Theoretical Radiation-Driven Winds from Stars and Discs
Mon. Not. R. Astron. Soc. 000, 000–000 (0000) Printed 17 July 2018 (MN LATEX style file v1.4) Comparison of theoretical radiation-driven winds from stars and discs. Daniel Proga Imperial College of Science, Technology and Medicine, Blackett Laboratory, Prince Consort Road, London SW7 2BZ, UK E-mail: [email protected] 17 July 2018 ABSTRACT We compare models of line-driven winds from accretion discs and single spherical stars. We look at the problem of scaling mass-loss rates and velocities of stellar and disc winds with model parameters. We find that stellar and disc winds driven by radiation, within the CAK framework, are very similar as far as mass-loss rates and velocities are concerned. Thus we can use analytic results for stellar winds to rescale, in a first order approximation, numerical results for disc winds. We also show how the CAK stellar solutions change when we take into account effects of very low luminosities or line-driving force. Key words: accretion discs – hydrodynamics – methods: numerical –stars: mass-loss – stars: early-type – galaxies: nuclei 1 INTRODUCTION der then, that radiation pressure has been proposed to power mass-loss also from accretion discs. Radiation pressure has been long recognised as effective at Despite a deep and quantitative understanding of powering mass-loss from luminous stars. It has been shown radiation-driven winds in stars it has not been straightfor- that stars with luminosities as low as 0.1 per cent of their ward to demonstrate that radiation can produce strong and Eddington limit can produce a powerful high velocity wind, fast winds from discs. -
The Fine Structure Constant and Electron (G-2) Factor Theory Review
4/26/2007 The Fine Structure Constant and Electron (g‐2) Factor Nick Hutzler Physics 135c Spring 2007 Theory Review • A (spin) magnetic moment μ quantifies how a particle interacts with magnetic fields • The electron has μ proportional to spin eh S S μ = g = gμB 2m h h • The fine structure constant α characterizes the strength of the EM interaction e2 1 α = ≈ 4πε0hc 137 Nick Hutzler // Ph 135c // Spring 2007 2 1 4/26/2007 Theory Review • The (spin) factor g is dimensionless and varies by partic le type – Classical charged sphere: g = 1 – Dirac point particle: g = 2 – Neutron: g = ‐3.826 085 45(90) – Proton: g = 5.585 694 713(46) • The anomalous magnetic moment is defined as a = (g‐2)/2 Nick Hutzler // Ph 135c // Spring 2007 3 Theory Review • Why measure g? – Probe of possible internal structure – One of best tests of QFT/QED, new physics – Fine structure constant α determined by g+QED Nick Hutzler // Ph 135c // Spring 2007 4 2 4/26/2007 Theory Review • Why isn’t g = 2? – Tree lllevel Feynman diagram gives g = 2 – Higher order corrections from quantum fluctuations add ~.001 Nick Hutzler // Ph 135c // Spring 2007 5 Theory Review • g and α are closely related – a has a series expansion in α (remember that α is commonly expanded in perturbation series) α α 2 α 3 a = C1()π + C2 ( π ) + C3 ( π ) +L+ aμτ + ahadronic + aweak – The Ci are calculated from QED – The aμτ,hadronic, weak terms are the QED muon/tau contribution, and non‐QED hadronic and weak contributions (respectively) Nick Hutzler // Ph 135c // Spring 2007 6 3 4/26/2007 Theory Review -
Astronomy Cast Episode 265 for Monday, May 14, 2012 Arthur Eddington
Astronomy Cast Episode 265 for Monday, May 14, 2012 Arthur Eddington Fraser: Welcome to Astronomy Cast, our weekly facts-based journey through the cosmos, where we help you understand not only what we know, but how we know what we know. My name is Fraser Cain; I’m the publisher of Universe Today, and with me is Dr. Pamela Gay, a professor at Southern Illinois University – Edwardsville. Hi, Pamela. How are you doing? Pamela: I’m doing well. How are you doing, Fraser? Fraser: Doing good. Now last week we asked nicely for donations and a bunch of you responded and that was fantastic, and I think we’re going to make this a two-parter, where we ask you for donations again. So if Astronomy Cast is important to you, and you want to help contribute to the show, we’ve got sort of editing people, transcript people, education outreach, and we really need your help. So you can go to Astrosphere.org and there’s a place to donate there, and also, actually you can actually donate from within Youtube as well, right? Pamela: Yeah, there’s a link on Youtube, and you can just go to Astronomycast.com and there’s a donations link there. We’ve done everything we can to make it easy for you to donate, so donate via Paypal either via the Astronomy Cast website, the Youtube website, or the Astrosphere New Media website. Fraser and I do this for the love of…well, actually, it’s more because you love it, and we’re trying to respond to your love, but we do have Preston, who we torture with our audio goofs, we have Joe, who’s helping to keep everything running, we have Nancy, who writes our show notes, we have Rome, who does all of our transcripts, and we want to feed our staff. -
A Note on the Fine Structure Constant
University of Nebraska - Lincoln DigitalCommons@University of Nebraska - Lincoln Sociology Department, Faculty Publications Sociology, Department of Spring 4-30-2020 A note on the fine structure constant Bilal Khan University of Nebraska-Lincoln, [email protected] Irshadullah Khan Quaid-i-Azam University, Islamabad, Pakistan, [email protected] Follow this and additional works at: https://digitalcommons.unl.edu/sociologyfacpub Part of the Other Mathematics Commons, and the Other Physics Commons Khan, Bilal and Khan, Irshadullah, "A note on the fine structure constant" (2020). Sociology Department, Faculty Publications. 705. https://digitalcommons.unl.edu/sociologyfacpub/705 This Article is brought to you for free and open access by the Sociology, Department of at DigitalCommons@University of Nebraska - Lincoln. It has been accepted for inclusion in Sociology Department, Faculty Publications by an authorized administrator of DigitalCommons@University of Nebraska - Lincoln. A note on the fine-structure constant arXiv:submit/3155246, currently "on hold" Bilal Khan∗ Irshadullah Khany Department of Computer Science and Sociology Department of Mathematics University of Nebraska-Lincoln Quaid-i-Azam University Lincoln, Nebraska, USA Islamabad, Pakistan [email protected] [email protected] Abstract We derive the numerical value of the fine structure constant α in purely number-theoretic terms, under the assumption that in a system of charges between two parallel conducting plates, the Casimir energy and the mutual Coulomb interaction energy agree. In 1916, based on Michelson and Morley’s precise measurement of the hydrogen atom spectrum [1], Arnold Sommerfeld extended the Bohr model to include elliptical orbits and relativistic dependence of mass on velocity, introducing the term α for the fine-structure constant [2].