On Development and Evolution of Trichoplax Adhaerens (Placozoa)
Total Page:16
File Type:pdf, Size:1020Kb
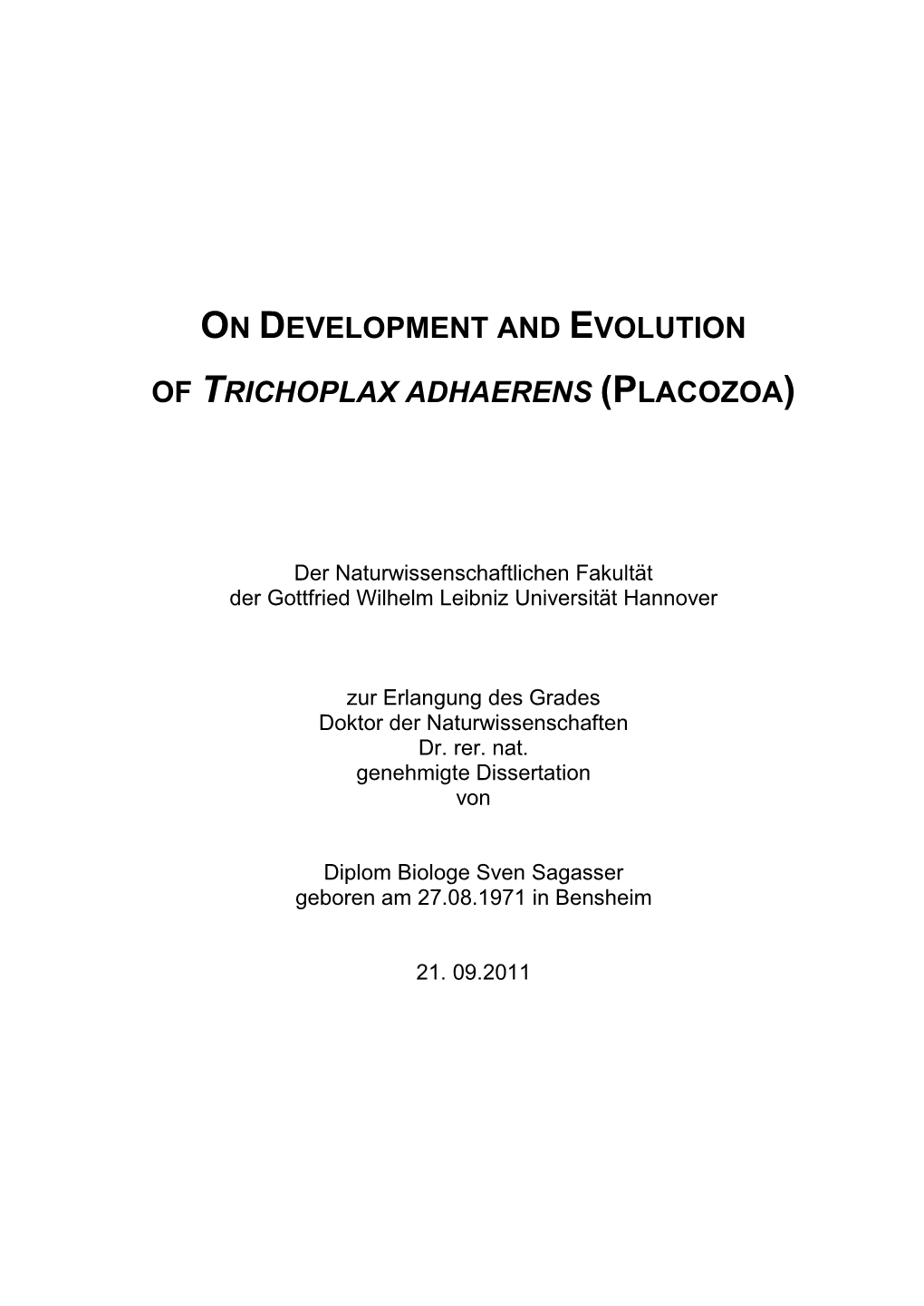
Load more
Recommended publications
-
Trends of Aquatic Alien Species Invasions in Ukraine
Aquatic Invasions (2007) Volume 2, Issue 3: 215-242 doi: http://dx.doi.org/10.3391/ai.2007.2.3.8 Open Access © 2007 The Author(s) Journal compilation © 2007 REABIC Research Article Trends of aquatic alien species invasions in Ukraine Boris Alexandrov1*, Alexandr Boltachev2, Taras Kharchenko3, Artiom Lyashenko3, Mikhail Son1, Piotr Tsarenko4 and Valeriy Zhukinsky3 1Odessa Branch, Institute of Biology of the Southern Seas, National Academy of Sciences of Ukraine (NASU); 37, Pushkinska St, 65125 Odessa, Ukraine 2Institute of Biology of the Southern Seas NASU; 2, Nakhimova avenue, 99011 Sevastopol, Ukraine 3Institute of Hydrobiology NASU; 12, Geroyiv Stalingrada avenue, 04210 Kiyv, Ukraine 4Institute of Botany NASU; 2, Tereschenkivska St, 01601 Kiyv, Ukraine E-mail: [email protected] (BA), [email protected] (AB), [email protected] (TK, AL), [email protected] (PT) *Corresponding author Received: 13 November 2006 / Accepted: 2 August 2007 Abstract This review is a first attempt to summarize data on the records and distribution of 240 alien species in fresh water, brackish water and marine water areas of Ukraine, from unicellular algae up to fish. A checklist of alien species with their taxonomy, synonymy and with a complete bibliography of their first records is presented. Analysis of the main trends of alien species introduction, present ecological status, origin and pathways is considered. Key words: alien species, ballast water, Black Sea, distribution, invasion, Sea of Azov introduction of plants and animals to new areas Introduction increased over the ages. From the beginning of the 19th century, due to The range of organisms of different taxonomic rising technical progress, the influence of man groups varies with time, which can be attributed on nature has increased in geometrical to general processes of phylogenesis, to changes progression, gradually becoming comparable in in the contours of land and sea, forest and dimensions to climate impact. -
New Zealand Oceanographic Institute Memoir 100
ISSN 0083-7903, 100 (Print) ISSN 2538-1016; 100 (Online) , , II COVER PHOTO. Dictyodendrilla cf. cavernosa (Lendenfeld, 1883) (type species of Dictyodendri/la Bergquist, 1980) (see page 24), from NZOI Stn I827, near Rikoriko Cave entrance, Poor Knights Islands Marine Reserve. Photo: Ken Grange, NZOI. This work is licensed under the Creative Commons Attribution-NonCommercial-NoDerivs 3.0 Unported License. To view a copy of this license, visit http://creativecommons.org/licenses/by-nc-nd/3.0/ NATIONAL INSTITUTE OF WATER AND ATMOSPHERIC RESEARCH The Marine Fauna of New Zealand: Index to the Fauna 2. Porifera by ELLIOT W. DAWSON N .Z. Oceanographic Institute, Wellington New Zealand Oceanographic Institute Memoir 100 1993 • This work is licensed under the Creative Commons Attribution-NonCommercial-NoDerivs 3.0 Unported License. To view a copy of this license, visit http://creativecommons.org/licenses/by-nc-nd/3.0/ Cataloguing in publication DAWSON, E.W. The marine fauna of New Zealand: Index to the Fauna 2. Porifera / by Elliot W. Dawson - Wellington: New Zealand Oceanographic Institute, 1993. (New Zealand Oceanographic Institute memoir, ISSN 0083-7903, 100) ISBN 0-478-08310-6 I. Title II. Series UDC Series Editor Dennis P. Gordon Typeset by Rose-Marie C. Thompson NIWA Oceanographic (NZOI) National Institute of Water and Atmospheric Research Received for publication: 17 July 1991 © NIWA Copyright 1993 2 This work is licensed under the Creative Commons Attribution-NonCommercial-NoDerivs 3.0 Unported License. To view a copy of this license, visit http://creativecommons.org/licenses/by-nc-nd/3.0/ CONTENTS Page ABSTRACT 5 INTRODUCTION 5 SCOPE AND ARRANGEMENT 7 SYSTEMATIC LIST 8 Class DEMOSPONGIAE 8 Subclass Homosclcromorpha .............................................................................................. -
Appendix: Some Important Early Collections of West Indian Type Specimens, with Historical Notes
Appendix: Some important early collections of West Indian type specimens, with historical notes Duchassaing & Michelotti, 1864 between 1841 and 1864, we gain additional information concerning the sponge memoir, starting with the letter dated 8 May 1855. Jacob Gysbert Samuel van Breda A biography of Placide Duchassaing de Fonbressin was (1788-1867) was professor of botany in Franeker (Hol published by his friend Sagot (1873). Although an aristo land), of botany and zoology in Gent (Belgium), and crat by birth, as we learn from Michelotti's last extant then of zoology and geology in Leyden. Later he went to letter to van Breda, Duchassaing did not add de Fon Haarlem, where he was secretary of the Hollandsche bressin to his name until 1864. Duchassaing was born Maatschappij der Wetenschappen, curator of its cabinet around 1819 on Guadeloupe, in a French-Creole family of natural history, and director of Teyler's Museum of of planters. He was sent to school in Paris, first to the minerals, fossils and physical instruments. Van Breda Lycee Louis-le-Grand, then to University. He finished traveled extensively in Europe collecting fossils, especial his studies in 1844 with a doctorate in medicine and two ly in Italy. Michelotti exchanged collections of fossils additional theses in geology and zoology. He then settled with him over a long period of time, and was received as on Guadeloupe as physician. Because of social unrest foreign member of the Hollandsche Maatschappij der after the freeing of native labor, he left Guadeloupe W etenschappen in 1842. The two chief papers of Miche around 1848, and visited several islands of the Antilles lotti on fossils were published by the Hollandsche Maat (notably Nevis, Sint Eustatius, St. -
Number of Living Species in Australia and the World
Numbers of Living Species in Australia and the World 2nd edition Arthur D. Chapman Australian Biodiversity Information Services australia’s nature Toowoomba, Australia there is more still to be discovered… Report for the Australian Biological Resources Study Canberra, Australia September 2009 CONTENTS Foreword 1 Insecta (insects) 23 Plants 43 Viruses 59 Arachnida Magnoliophyta (flowering plants) 43 Protoctista (mainly Introduction 2 (spiders, scorpions, etc) 26 Gymnosperms (Coniferophyta, Protozoa—others included Executive Summary 6 Pycnogonida (sea spiders) 28 Cycadophyta, Gnetophyta under fungi, algae, Myriapoda and Ginkgophyta) 45 Chromista, etc) 60 Detailed discussion by Group 12 (millipedes, centipedes) 29 Ferns and Allies 46 Chordates 13 Acknowledgements 63 Crustacea (crabs, lobsters, etc) 31 Bryophyta Mammalia (mammals) 13 Onychophora (velvet worms) 32 (mosses, liverworts, hornworts) 47 References 66 Aves (birds) 14 Hexapoda (proturans, springtails) 33 Plant Algae (including green Reptilia (reptiles) 15 Mollusca (molluscs, shellfish) 34 algae, red algae, glaucophytes) 49 Amphibia (frogs, etc) 16 Annelida (segmented worms) 35 Fungi 51 Pisces (fishes including Nematoda Fungi (excluding taxa Chondrichthyes and (nematodes, roundworms) 36 treated under Chromista Osteichthyes) 17 and Protoctista) 51 Acanthocephala Agnatha (hagfish, (thorny-headed worms) 37 Lichen-forming fungi 53 lampreys, slime eels) 18 Platyhelminthes (flat worms) 38 Others 54 Cephalochordata (lancelets) 19 Cnidaria (jellyfish, Prokaryota (Bacteria Tunicata or Urochordata sea anenomes, corals) 39 [Monera] of previous report) 54 (sea squirts, doliolids, salps) 20 Porifera (sponges) 40 Cyanophyta (Cyanobacteria) 55 Invertebrates 21 Other Invertebrates 41 Chromista (including some Hemichordata (hemichordates) 21 species previously included Echinodermata (starfish, under either algae or fungi) 56 sea cucumbers, etc) 22 FOREWORD In Australia and around the world, biodiversity is under huge Harnessing core science and knowledge bases, like and growing pressure. -
Bio 002: Invertebrate Biology Phylum Coelenterata
BIO 002: INVERTEBRATE BIOLOGY PHYLUM COELENTERATA PHYLUM COELENTERATA (CNIDERIA) The coelenterates include more than 9000 living species. They are all aquatic, mostly marine but also with a few fresh water forms. They are often found abundantly in warm temperature or subtropical waters. These animals are carnivores, they actively do not move from place to place, rather they lie in wait and capture their prey (e.g. fishes, crustaceans) with the tentacles that ring their mouth. Examples include jelly fishes, sea anemones, corals, hydroids etc. Characteristic Features of Coelenterates Coelenterates are multicellular organisms They have tissue-grade of organization All members of this phylum are aquatic animals They are radially symmetrical or the symmetry of a wheel The body wall is diploblastic. It is made up of two layers of cells, namely the ectoderm and the endoderm with a non–cellular layer called mesogloea in between They have no coelom, hence coelenterates are acoelomate animals Two individual forms of coelenterate exist: polyps and medusa Respiratory, excretory and circulatory system are absent Life history has alternation of generations or metagenesis. Forms of Coelenterate Coelenterates may have two basic forms which are: Polyp Medusa Polyps are cylindrical and are usually found attached to a firm substrate. They may be solitary or colonial. In a polyp, the mouth faces away from the substrate on which the animal is growing and is therefore often facing upwards. The polyp is the sedentary, benthic form In contrast, most Medusa free-floating and are often umbrella shaped with their mouth usually pointing downwards and the tentacles hanging down around them. -
Simple Animals Sponges and Placozoa the Twig of the Tree That Is the Animals the Tour Begins
Animal Kingdom Simple animals Sponges and placozoa Tom Hartman Asymmetry www.tuatara9.co.uk Animal form and function 1 Module 111121 2 The twig of the tree that is the animals Animals All other animals Animals Sponges Choanoflagellates Choanoflagellates Fungi Fungi 3 4 All other animals Animals (bilateralia) Radiata Sponges Choanoflagellates Fungi The tour begins 5 6 1 Animal Kingdom The Phylum Quirky phyla • In the standard Linnean system (and taxonomic systems • There are 39 animal phyla (+/- 10!) based on it), a Phylum is the taxonomic category between Kingdom and Class. • The Micrognathozoa were • A phylum is a major ranking of organisms, defined discovered in 2000 (1 species)in according to the most basic body-parts shared by that springs in Greenland. group. But we must include the creatures and their • Xenoturbellida removed from the common ancestor. molluscs and moved to the – Chordata (animals with a notochord - vertebrates and others), – Arthropoda (animals with a jointed exoskeleton) dueterostomes when DNA – Mollusca (animals with a shell-secreting mantle), evidence was discounted due to – Angiosperma (flowering plants), and so on. what it had eaten! – A number of traditional Phyla - e.g. Protozoa, possibly Arthropoda - • Cycliophora discovered on the are probably invalid (polyphyletic). lips of lobsters in 1995. 7 8 The Class Our journey • In the Linnean system (and taxonomic systems Kingdom Group Phylum based on it), a Class is the taxonomic category Porifera between Phylum and Order. Placozoa • A class is a major group of organisms, e.g. Cnidaria Mammalia, Gastropoda, Insecta, etc that Parazoa Ctenophora contains a large number of different Radiata Platyhelminthes sublineages, but have shared characteristics in Animalia Protostome Rotifera common e.g. -
FAU Institutional Repository
FAU Institutional Repository http://purl.fcla.edu/fau/fauir This paper was submitted by the faculty of FAU’s Harbor Branch Oceanographic Institute. Notice: ©1999 Academic Press. This manuscript is an author version with the final publication available and may be cited as: Young, C. M. (1999). Marine invertebrate larvae. In E. Knobil & J. D. Neill (eds.), Encyclopedia of Reproduction, 3. (pp. 89-97). London, England, and San Diego, CA: Academic Press. --------1111------- Marine Invertebrate Larvae Craig M. Young Harbor Branch Oceanographic Institution 1. What Is a Larva? metamorphOSiS Morphological and physiological changes II. The Production of Larvae that occur during the transition from the larval phase to iII. Larval forms and Diversity the juvenile phase: often coincides with settlement in ben IV. Larval Feeding and Nutrition thic species. V. Larval Orientation, Locomotion, Dispersal, and mixed development A developmental mode that includes a Mortality brooded or encapsulated embryonic stage as well as a free VI. Larval Settlement and Metamorphosis swimming larval stage. VlI. Ecological and Evolutionary Significance of Larvae planktotrophic larva A feeding larva that obtains at least part VlIl. Economic and Medical Importance of Larvae of its nutritional needs from either particulate or dissolved exogenous sources. Planktotrophic larvae generally hatch from small, transparent eggs. GLOSSARY settlement The permanent transition of a larva from the plankton to the benthos. In sessile organisms, settlement atrochal larva A uniformly ciliated larva (cilia not arranged is marked by adhesion to the substratum. It is often closely in distinct bands). associated with metamorphosis and may involve habitat se competent larva A larva that is physiologically and morpho lection. -
Metazoa Based on How Organized They Are
BIOLOGY 18: Phyla of the “Changed Animals” Climbing the evolutionary lad- der from the protozoa we find higher levels of organization. Organisms are grouped into mesozoa and metazoa based on how organized they are. The simplest multicellular organisms (those having many cells) are the me- sozoa (“middle animals”). These or- ganisms are simple parasitic worms. Parasitic means that they live at the expense of some other organism. They often suck nutrient-rich fluids right out of the other organism, but they don’t usually kill the organism or they lose their source of food. The metazoa, meaning “changed animals” can be larger in size because they have different kinds of cells that work together to bring things in, take things out, protect the whole organ- ism, and perform other duties that enable them to live in a wider range of habitats. Because of the various cell types, organisms at this level be- gin taking on a variety of shapes that are not possible among colonies of identical cells. The metazoa include all other phyla of animals from the simple to the complex. A strawberry sponge of the phylum Porifera. Kingdom Animalia: Metazoa Kingdom Porifera Coelenterata Ctenophora Platyhelminthes Rhinochocoela Nematoda Acanthocephala Chaetognatha Nematomorpha Hemichordata (sponges) (flat worms) (proboscis, (round (spiny-headed (arrow (horsehair (acorn worms) nemertine & worms) worms) worms) worms) Phylum ribbon worms) 186 BIOLOGY The next set of organisms in terms of their simplicity is the phylum Porifera—the sponges. There are many types of these animals that live in the sea and a few that live in fresh water. -
OREGON ESTUARINE INVERTEBRATES an Illustrated Guide to the Common and Important Invertebrate Animals
OREGON ESTUARINE INVERTEBRATES An Illustrated Guide to the Common and Important Invertebrate Animals By Paul Rudy, Jr. Lynn Hay Rudy Oregon Institute of Marine Biology University of Oregon Charleston, Oregon 97420 Contract No. 79-111 Project Officer Jay F. Watson U.S. Fish and Wildlife Service 500 N.E. Multnomah Street Portland, Oregon 97232 Performed for National Coastal Ecosystems Team Office of Biological Services Fish and Wildlife Service U.S. Department of Interior Washington, D.C. 20240 Table of Contents Introduction CNIDARIA Hydrozoa Aequorea aequorea ................................................................ 6 Obelia longissima .................................................................. 8 Polyorchis penicillatus 10 Tubularia crocea ................................................................. 12 Anthozoa Anthopleura artemisia ................................. 14 Anthopleura elegantissima .................................................. 16 Haliplanella luciae .................................................................. 18 Nematostella vectensis ......................................................... 20 Metridium senile .................................................................... 22 NEMERTEA Amphiporus imparispinosus ................................................ 24 Carinoma mutabilis ................................................................ 26 Cerebratulus californiensis .................................................. 28 Lineus ruber ......................................................................... -
Animal Evolution: Looking for the First Nervous System
Dispatch R655 cellular or symbiotic functions, cannot genome reduction in the symbiont, 10. Nakabachi, A., Ishida, K., Hongoh, Y., Ohkuma, M., and Miyagishima, S. (2014). be lost without harmful or fatal results. HGT from various sources to the host Aphid gene of bacterial origin encodes a But these genes can be replaced, genome to maintain symbiont function, protein transported to an obligate and perhaps ever more easily as and now the targeting of protein endosymbiont. Curr. Biol. 24, R640–R641. 11. Moran, N.A., and Jarvik, T. (2010). Lateral the interaction networks of the products from host to symbiont has transfer of genes from fungi underlies endosymbiont reduce in complexity, even been found [4,10]. These make carotenoid production in aphids. Science 328, 624–627. thus reducing pressures on proteins clean separation of endosymbiont from 12. Jorgenson, M.A., Chen, Y., Yahashiri, A., to co-evolve. In a sense, when genes organelle more difficult to see, Popham, D.L., and Weiss, D.S. (2014). The are transferred from symbiont or prompting us not to look for the point bacterial septal ring protein RlpA is a lytic transglycosylase that contributes to rod organelle to the host nuclear genome when a symbiont ‘becomes’ an shape and daughter cell separation in and their proteins targeted back, this is organelle, but rather to ask, ‘Is there Pseudomonas aeruginosa. Mol. Microbiol. 93, 113–128. a compensatory change [4,9,14,17]. really anything so special about 13. Acuna, R., Padilla, B.E., Florez-Ramos, C.P., But other compensatory transfers that organelles?’ Rubio, J.D., Herrera, J.C., Benavides, P., affect a symbiont or organelle can also Lee, S.J., Yeats, T.H., Egan, A.N., Doyle, J.J., et al. -
Stylohates: a Shell-Forming Sea Anemone (Coelenterata, Anthozoa, Actiniidae)1
Pacific Science (1980), vol. 34, no. 4 © 1981 by The University Press of Hawaii. All rights reserved Stylohates: A Shell-Forming Sea Anemone (Coelenterata, Anthozoa, Actiniidae) 1 DAPHNE FAUTIN DUNN,2 DENNIS M. DEVANEY,3 and BARRY ROTH 4 ABSTRACT: Anatomy and cnidae distinguish two species of deep-sea ac tinians that produce coiled, chitinous shells inhabited by hermit crabs of the genus Parapagurus. The actinian type species, Stylobates aeneus, first assigned to the Mollusca, occurs around Hawaii and Guam with P. dofleini. Stylobates cancrisocia, originally described as Isadamsia cancrisocia, occurs off east Africa with P. trispinosus. MANY MEMBERS OF THE ORDER Actiniaria pedal disk secretes a chitinous cuticle over attach obligately or facultatively to gas the small mollusk shell which the pagurid tropod shells inhabited by hermit crabs. had initially occupied and to which the small Some of these partnerships seem to be actinian had first attached, often extending strictly phoretic, the normally sedentary sea the cuticular material beyond the lip of the anemone being transported by the motile shell (Balss 1924, Faurot 1910, Gosse 1858). hermit crab (Ross 1971, 1974b). The re This arrangement affords the crab mainly lationships between other species pairs are mechanical protection (Ross 1971). mutualistic, the anemone gaining motility Carlgren (I928a) described as a new genus while protecting its associate from predation and species Isadamsia cancrisocia (family (Balasch and Mengual 1974; Hand 1975; Actiniidae), an actinian attached to a shell McLean and Mariscal 1973; Ross 1971, occupied by a hermit crab, from four speci 1974b; Ross and von Boletsky 1979). As the mens collected by the Deutschen Tiefsee crustacean grows, it must move to increas Expedition (1898-1899) at 818 m in the ingly larger shells. -
Basal Metazoans - Dirk Erpenbeck, Simion Paul, Michael Manuel, Paulyn Cartwright, Oliver Voigt and Gert Worheide
EVOLUTION OF PHYLOGENETIC TREE OF LIFE - Basal Metazoans - Dirk Erpenbeck, Simion Paul, Michael Manuel, Paulyn Cartwright, Oliver Voigt and Gert Worheide BASAL METAZOANS Dirk Erpenbeck Ludwig-Maximilians Universität München, Germany Simion Paul and Michaël Manuel Université Pierre et Marie Curie in Paris, France. Paulyn Cartwright University of Kansas USA. Oliver Voigt and Gert Wörheide Ludwig-Maximilians Universität München, Germany Keywords: Metazoa, Porifera, sponges, Placozoa, Cnidaria, anthozoans, jellyfishes, Ctenophora, comb jellies Contents 1. Introduction on ―Basal Metazoans‖ 2. Phylogenetic relationships among non-bilaterian Metazoa 3. Porifera (Sponges) 4. Placozoa 5. Ctenophora (Comb-jellies) 6. Cnidaria 7. Cultural impact and relevance to human welfare Glossary Bibliography Biographical Sketch Summary Basal metazoans comprise the four non-bilaterian animal phyla Porifera (sponges), Cnidaria (anthozoans and jellyfishes), Placozoa (Trichoplax) and Ctenophora (comb jellies). The phylogenetic position of these taxa in the animal tree is pivotal for our understanding of the last common metazoan ancestor and the character evolution all Metazoa,UNESCO-EOLSS but is much debated. Morphological, evolutionary, internal and external phylogenetic aspects of the four phyla are highlighted and discussed. SAMPLE CHAPTERS 1. Introduction on “Basal Metazoans” In many textbooks the term ―lower metazoans‖ still refers to an undefined assemblage of invertebrate phyla, whose phylogenetic relationships were rather undefined. This assemblage may contain both bilaterian and non-bilaterian taxa. Currently, ―Basal Metazoa‖ refers to non-bilaterian animals only, four phyla that lack obvious bilateral symmetry, Porifera, Placozoa, Cnidaria and Ctenophora. ©Encyclopedia of Life Support Systems (EOLSS) EVOLUTION OF PHYLOGENETIC TREE OF LIFE - Basal Metazoans - Dirk Erpenbeck, Simion Paul, Michael Manuel, Paulyn Cartwright, Oliver Voigt and Gert Worheide These four phyla have classically been known as ―diploblastic‖ Metazoa.