1 a Genetic Perspective on the Domestication Continuum Laurent
Total Page:16
File Type:pdf, Size:1020Kb
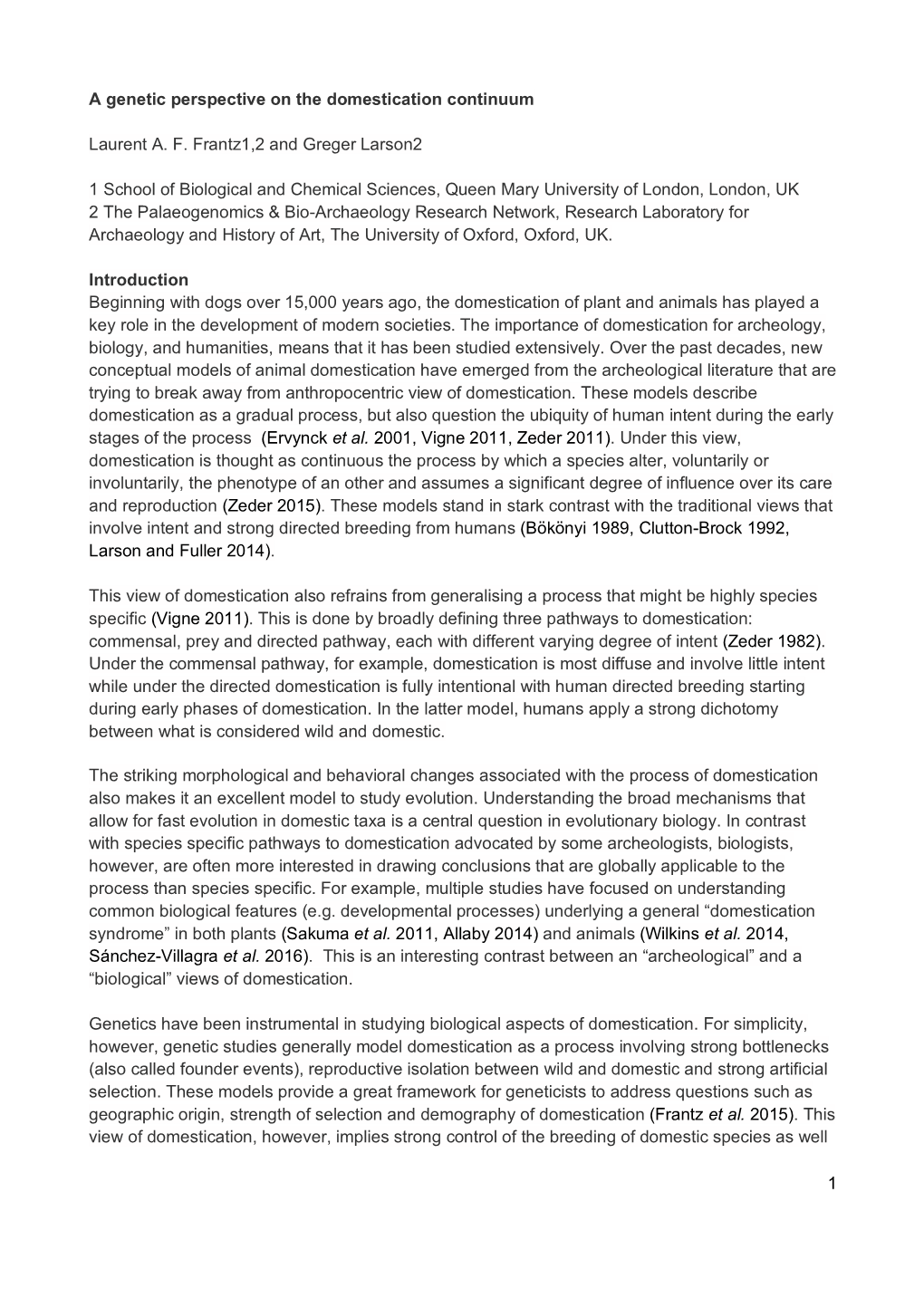
Load more
Recommended publications
-
The Evolution of Animal Domestication
See discussions, stats, and author profiles for this publication at: https://www.researchgate.net/publication/266740619 The Evolution of Animal Domestication Article in Annual Review of Ecology Evolution and Systematics · October 2014 DOI: 10.1146/annurev-ecolsys-120213-091620 CITATIONS READS 179 3,162 2 authors: Greger Larson Dorian Q Fuller University of Oxford University College London 196 PUBLICATIONS 6,523 CITATIONS 322 PUBLICATIONS 12,021 CITATIONS SEE PROFILE SEE PROFILE Some of the authors of this publication are also working on these related projects: Dog Domestication View project Rwandan Archaeology View project All content following this page was uploaded by Dorian Q Fuller on 12 October 2014. The user has requested enhancement of the downloaded file. ES45CH06-Larson ARI 16 September 2014 11:18 V I E E W R S I E N C N A D V A The Evolution of Animal Domestication Greger Larson1 and Dorian Q. Fuller2 1Durham Evolution and Ancient DNA, Department of Archaeology, Durham University, Durham, DH1 3LE, United Kingdom; email: [email protected] 2Institute of Archaeology, University College London, London WC1H 0PY, United Kingdom Annu. Rev. Ecol. Evol. Syst. 2014. 66:115–36 Keywords The Annual Review of Ecology, Evolution, and archaeology, genetics, livestock, introgression, selection, agriculture Systematics is online at ecolsys.annualreviews.org This article’s doi: Abstract 10.1146/annurev-ecolsys-120213-091620 The domestication of plants and animals over the past 11,500 years has Copyright c 2014 by Annual Reviews. had a significant effect not just on the domesticated taxa but also on human All rights reserved evolution and on the biosphere as a whole. -
Archaeological Central American Maize Genomes Suggest Ancient Gene Flow from South America
Archaeological Central American maize genomes suggest ancient gene flow from South America Logan Kistlera,1, Heather B. Thakarb, Amber M. VanDerwarkerc, Alejandra Domicd,e, Anders Bergströmf, Richard J. Georgec, Thomas K. Harperd, Robin G. Allabyg, Kenneth Hirthd, and Douglas J. Kennettc,1 aDepartment of Anthropology, National Museum of Natural History, Smithsonian Institution, Washington, DC 20560; bDepartment of Anthropology, Texas A&M University, College Station, TX 77843; cDepartment of Anthropology, University of California, Santa Barbara, CA 93106; dDepartment of Anthropology, The Pennsylvania State University, University Park, PA 16802; eDepartment of Geosciences, The Pennsylvania State University, University Park, PA 16802; fAncient Genomics Laboratory, The Francis Crick Institute, NW1 1AT London, United Kingdom; and gSchool of Life Sciences, University of Warwick, CV4 7AL Coventry, United Kingdom Edited by David L. Lentz, University of Cincinnati, Cincinnati, OH, and accepted by Editorial Board Member Elsa M. Redmond November 3, 2020 (received for review July 24, 2020) Maize (Zea mays ssp. mays) domestication began in southwestern 16). However, precolonial backflow of divergent maize varieties Mexico ∼9,000 calendar years before present (cal. BP) and humans into Central and Mesoamerica during the last 9,000 y remains dispersed this important grain to South America by at least 7,000 understudied, and could have ramifications for the history of cal. BP as a partial domesticate. South America served as a second- maize as a staple in the region. ary improvement center where the domestication syndrome be- Morphological evidence from ancient maize found in ar- came fixed and new lineages emerged in parallel with similar chaeological sites combined with DNA data confirms a complex processes in Mesoamerica. -
The History of Farm Foxes Undermines the Animal Domestication Syndrome, Trends in Ecology & Evolution (2019)
Please cite this article in press as: Lord et al., The History of Farm Foxes Undermines the Animal Domestication Syndrome, Trends in Ecology & Evolution (2019), https://doi.org/10.1016/j.tree.2019.10.011 Trends in Ecology & Evolution Opinion The History of Farm Foxes Undermines the Animal Domestication Syndrome Kathryn A. Lord,1,2 Greger Larson,3,@ Raymond P. Coppinger,4,6 and Elinor K. Karlsson1,2,5,@,* The Russian Farm-Fox Experiment is the best known experimental study in animal domestication. Highlights By subjecting a population of foxes to selection for tameness alone, Dimitry Belyaev generated The ‘domestication syndrome’ has foxes that possessed a suite of characteristics that mimicked those found across domesticated been a central focus of research species. This ‘domestication syndrome’ has been a central focus of research into the biological into the biological processes un- pathways modified during domestication. Here, we chart the origins of Belyaev’s foxes in derlying domestication. The eastern Canada and critically assess the appearance of domestication syndrome traits across an- Russian Farm-Fox Experiment was imal domesticates. Our results suggest that both the conclusions of the Farm-Fox Experiment the first to test whether there is a and the ubiquity of domestication syndrome have been overstated. To understand the process causal relationship between selec- tion for tameness and the domes- of domestication requires a more comprehensive approach focused on essential adaptations to tication syndrome. human-modified environments. Historical records and genetic The Origins of Domestication Syndrome analysis show that the foxes used in The domestication syndrome describes a suite of behavioral and morphological characteristics the Farm-Fox Experiment origi- consistently observed in domesticated populations. -
Hered 445 Master..Hered 445 .. Page648
Heredity 81 (1998) 648–658 Received 19 March 1998, accepted 15 June 1998 Genetic analysis of the domestication syndrome in pearl millet (Pennisetum glaucum L., Poaceae): inheritance of the major characters V. PONCET*%, F. LAMY%, J. ENJALBERT^, H. JOLY§, A. SARR% & T. ROBERT% %Laboratoire d’Evolution et Syst´ematique, Universit´e Paris XI, Bˆat. 362, F-91405 Orsay Cedex, France, ^Station de G´en´etique V´eg´etale, Ferme du Moulon, F-91190 Gif sur Yvette, France and §CIRAD-Forˆet, campus international de Baillarguet, BP 5035, F-34032 Montpellier Cedex 1, France The inheritance of domestication traits distinguishing pearl millet (Pennisetum glaucum) from its wild relatives (P. mollissimum) was assessed in F2 progenies derived from a cross between a typical landrace of pearl millet and a wild ecotype. Despite a high level of recombination between the two genomes, the existence of preferential associations between some characters was demonstrated, leading, in particular, to cultivated-like phenotypes. Traits determining spikelet structure showed simple Mendelian inheritance. Moreover, the genes encoding these traits mapped in a linkage group where quantitative trait loci for spike size and tillering habit were found. This linkage group could correspond to one of the two chromosome segments that have already been shown to be involved in the variation for spikelet structure in progenies from several cultivatedÅwild crosses. A synthetic map of these two regions is given. The evolutionary significance of this genomic organization in relation to the domestication process is discussed, as well as its potential use for pearl millet genetic resources enhancement. Keywords: domestication, genetic map, pearl millet, Pennisetum glaucum. -
Paleogenomics of Animal Domestication
Paleogenomics of Animal Domestication Evan K. Irving-Pease, Hannah Ryan, Alexandra Jamieson, Evangelos A. Dimopoulos, Greger Larson, and Laurent A. F. Frantz Abstract Starting with dogs, over 15,000 years ago, the domestication of animals has been central in the development of modern societies. Because of its importance for a range of disciplines – including archaeology, biology and the humanities – domestication has been studied extensively. This chapter reviews how the field of paleogenomics has revolutionised, and will continue to revolutionise, our under- standing of animal domestication. We discuss how the recovery of ancient DNA from archaeological remains is allowing researchers to overcome inherent shortcom- ings arising from the analysis of modern DNA alone. In particular, we show how DNA, extracted from ancient substrates, has proven to be a crucial source of information to reconstruct the geographic and temporal origin of domestic species. We also discuss how ancient DNA is being used by geneticists and archaeologists to directly observe evolutionary changes linked to artificial and natural selection to generate a richer understanding of this fascinating process. Keywords Ancient DNA · Archaeology · Domestication · Entomology · Evolution · Genomics · Zoology E. K. Irving-Pease (*) · H. Ryan · A. Jamieson · E. A. Dimopoulos · G. Larson The Palaeogenomics and Bio-Archaeology Research Network, Research Laboratory for Archaeology and History of Art, University of Oxford, Oxford, UK e-mail: [email protected] L. A. F. Frantz (*) The Palaeogenomics and Bio-Archaeology Research Network, Research Laboratory for Archaeology and History of Art, University of Oxford, Oxford, UK School of Biological and Chemical Sciences, Queen Mary University of London, London, UK e-mail: [email protected] Charlotte Lindqvist and Om P. -
Awn Reduction and the Domestication of Asian Rice: a Syndrome Or Crop Improvement Trait? Serge Svizzero, Avik Ray, Debarati Chakraborty
Awn Reduction and the Domestication of Asian Rice: A Syndrome or Crop Improvement Trait? Serge Svizzero, Avik Ray, Debarati Chakraborty To cite this version: Serge Svizzero, Avik Ray, Debarati Chakraborty. Awn Reduction and the Domestication of Asian Rice: A Syndrome or Crop Improvement Trait?. Economic Botany, Springer Verlag, 2019, pp.1-12. 10.1007/s12231-019-09465-0. hal-02275855 HAL Id: hal-02275855 https://hal.univ-reunion.fr/hal-02275855 Submitted on 2 Sep 2019 HAL is a multi-disciplinary open access L’archive ouverte pluridisciplinaire HAL, est archive for the deposit and dissemination of sci- destinée au dépôt et à la diffusion de documents entific research documents, whether they are pub- scientifiques de niveau recherche, publiés ou non, lished or not. The documents may come from émanant des établissements d’enseignement et de teaching and research institutions in France or recherche français ou étrangers, des laboratoires abroad, or from public or private research centers. publics ou privés. 1 Awn Reduction and the Domestication of Asian Rice: A Syndrome or Crop Improvement Trait? Serge Svizzero1, Avik Ray2,*, Debarati Chakraborty2,3 1- Faculté de Droit et d’Economie, Université de La Réunion, 15 Avenue René Cassin. CS 92003, 97744 Saint Denis Cedex 9, France, Tel: +262 262 13 82 58; ORCID: 0000-0003- 3895-7273 2 - Center for studies in Ethnobiology, Biodiversity, and sustainability (CEiBa), B.G. Road, Mokdumpur, Malda – 732103, West Bengal, India; ORCID: 0000-0003-1662-7679 3 - Department of Molecular Biology and Biotechnology, University of Kalyani, Kalyani, India; ORCID: 0000-0002-1939-9889 * [email protected] Running title: SVIZZERO, RAY AND CHAKRABORTY: RICE AWN REDUCTION Words account: 7596; Abstract: 180. -
(12) United States Patent (10) Patent No.: US 7.923,221 B1 Cabilly Et Al
US007.923221B1 (12) United States Patent (10) Patent No.: US 7.923,221 B1 Cabilly et al. (45) Date of Patent: *Apr. 12, 2011 (54) METHODS OF MAKING ANTIBODY HEAVY 4,512.922 A 4, 1985 Jones et al. AND LIGHT CHAINS HAVING SPECIFICITY 4,518,584 A 5, 1985 Mark 4,565,785 A 1/1986 Gilbert et al. FORADESIRED ANTIGEN 4,599,197 A 7, 1986 Wetzel 4,634,665 A 1/1987 Axel et al. (75) Inventors: Shmuel Cabilly, Monrovia, CA (US); 4,642,334 A 2f1987 Moore et al. Herbert L. Heyneker, Burlingame, CA 4,668,629 A 5/1987 Kaplan 4,704,362 A 11/1987 Itakura et al. (US); William E. Holmes, Pacifica, CA 4,713,339 A 12/1987 Levinson et al. (US); Arthur D. Riggs, LaVerne, CA 4,766,075 A 8, 1988 Goeddeletal. (US); Ronald B. Wetzel, San Francisco, 4,792.447 A 12/1988 Uhr et al. CA (US) 4,816,397 A * 3/1989 Boss et al. ...................... 435/68 4,816,567 A 3/1989 Cabilly et al. (73) Assignees: Genentech, Inc, South San Francisco, 4,965,196 A 10, 1990 Levinson et al. 5,081,235 A 1/1992 Shively et al. CA (US); City of Hope, Duarte, CA 5,098,833. A 3/1992 Lasky et al. (US) 5,116,964 A 5/1992 Capon et al. 5,137,721 A 8, 1992 Dallas (*) Notice: Subject to any disclaimer, the term of this 5,149,636 A 9, 1992 Axel et al. patent is extended or adjusted under 35 5,179,017 A 1/1993 Axel et al. -
How the Techniques of Molecular Biology Are Developed from Natural Systems
How the techniques of molecular biology are developed from natural systems Isobel Ronai [email protected] School of Life and Environmental Sciences, The University of Sydney, Sydney, NSW, Australia. Abstract A striking characteristic of the highly successful techniques in molecular biology is that they are derived from natural systems. RNA interference (RNAi), for example, utilises a mechanism that evolved in eukaryotes to destroy foreign nucleic acid. Other examples include restriction enzymes, the polymerase chain reaction, green fluorescent protein and CRISPR-Cas. I propose that biologists exploit natural molecular mechanisms for their effectors’ (protein or nucleic acid) activity and biological specificity (protein or nucleic acid can cause precise reactions). I also show that the developmental trajectory of novel techniques in molecular biology, such as RNAi, is four characteristic phases. The first phase is discovery of a biological phenomenon, typically as curiosity driven research. The second is identification of the mechanism’s trigger(s), the effector and biological specificity. The third is the application of the technique. The final phase is the maturation and refinement of the molecular biology technique. The development of new molecular biology techniques from nature is crucial for biological research. These techniques transform scientific knowledge and generate new knowledge. Keywords: mechanism; experiment; specificity; scientific practice; PCR; GFP. 1 Introduction Molecular biology is principally concerned with explaining the complex molecular phenomena underlying living processes by identifying the mechanisms that produce such processes (Tabery et al. 2015). In order to access the causal structure of molecular mechanisms it is generally necessary to manipulate the components of the mechanism and to observe the resulting effects with sophisticated molecular techniques. -
Were Ancestral Proteins Less Specific?
bioRxiv preprint doi: https://doi.org/10.1101/2020.05.27.120261; this version posted May 30, 2020. The copyright holder for this preprint (which was not certified by peer review) is the author/funder, who has granted bioRxiv a license to display the preprint in perpetuity. It is made available under aCC-BY-ND 4.0 International license. Were ancestral proteins less specific? 1 1,2,3 1,2* Lucas C. Wheeler and Michael J. Harms 2 1. Institute of Molecular Biology, University of Oregon, Eugene OR 97403 3 2. Department of Chemistry and Biochemistry, University of Oregon, Eugene OR 97403 4 3. Department of Ecology and Evolutionary Biology, University of Colorado, Boulder 5 CO 80309 6 7 1 bioRxiv preprint doi: https://doi.org/10.1101/2020.05.27.120261; this version posted May 30, 2020. The copyright holder for this preprint (which was not certified by peer review) is the author/funder, who has granted bioRxiv a license to display the preprint in perpetuity. It is made available under aCC-BY-ND 4.0 International license. Abstract 8 Some have hypothesized that ancestral proteins were, on average, less specific than their 9 descendants. If true, this would provide a universal axis along which to organize protein 10 evolution and suggests that reconstructed ancestral proteins may be uniquely powerful tools 11 for protein engineering. Ancestral sequence reconstruction studies are one line of evidence 12 used to support this hypothesis. Previously, we performed such a study, investigating the 13 evolution of peptide binding specificity for the paralogs S100A5 and S100A6. -
1 the Limits of Selection Under Plant Domestication Robin G Allaby1
The limits of selection under plant domestication Robin G Allaby1* ,Dorian Q Fuller2, James L Kitchen1, 3 1. School of Life Sciences, Gibbet Hill Campus, University of Warwick, Coventry CV4 7AL 2. Institute of Archaeology, University College London, 31-34 Gordon Square, London WC1H 0PY 3. Computational and Systems Biology, Rothamsted, Harpenden, Herts. * corresponding author email: [email protected] 1 Abstract Plant domestication involved a process of selection through human agency of a series of traits collectively termed the domestication syndrome. Current debate concerns the pace at which domesticated plants emerged from cultivated wild populations and how many genes were involved. Here we present simulations that test how many genes could have been involved by considering the cost of selection. We demonstrate the selection load that can be endured by populations increases with decreasing selection coefficients and greater numbers of loci down to values of about s = 0.005, causing a driving force that increases the number of loci under selection. As the number of loci under selection increases, an effect of co-selection increases resulting in individual unlinked loci being fixed more rapidly in out-crossing populations, representing a second driving force to increase the number of loci under selection. In inbreeding systems co-selection results in interference and reduced rates of fixation but does not reduce the size of the selection load that can be endured. These driving forces result in an optimum pace of genome evolution in which 50-100 loci are the most that could be under selection in a cultivation regime. Furthermore, the simulations do not preclude the existence of selective sweeps but demonstrate that they come at a cost of the selection load that can be endured and consequently a reduction of the capacity of plants to adapt to new environments, which may contribute to the explanation of why selective sweeps have been so rarely detected in genome studies. -
The Molecular Basis of Kale Domestication: Transcription Profiling of Leaves
bioRxiv preprint doi: https://doi.org/10.1101/2020.11.25.398347; this version posted December 2, 2020. The copyright holder for this preprint (which was not certified by peer review) is the author/funder, who has granted bioRxiv a license to display the preprint in perpetuity. It is made available under aCC-BY-NC-ND 4.0 International license. Arias et al., p. 1 1 2 Running head: Kale domestication 3 4 The molecular basis of Kale domestication: Transcription profiling of leaves 5 and meristems provides new insights into the evolution of a Brassica oleracea 6 vegetative morphotype 7 8 Tatiana Arias1,2,4*, Chad Niederhuth1,3,4, Paula McSteen1, J. Chris Pires1 9 10 11 1 Division of Biological Sciences, University of Missouri, Columbia, MO, United States 12 2 Current address: Tecnológico de Antioquia, Medellín, Colombia. 13 3 Current address: Department of Plant Biology, Michigan State University, East Lansing MI, 14 United States 15 4 Both authors contributed equally to this manuscript 16 17 *author for correspondence: [email protected] 18 19 Manuscript received _______; revision accepted _______. 20 Short title: Kale domestication 21 22 bioRxiv preprint doi: https://doi.org/10.1101/2020.11.25.398347; this version posted December 2, 2020. The copyright holder for this preprint (which was not certified by peer review) is the author/funder, who has granted bioRxiv a license to display the preprint in perpetuity. It is made available under aCC-BY-NC-ND 4.0 International license. Arias et al., p. 2 23 ABSTRACT [237 words - 250 max] 24 Morphotypes of Brassica oleracea are the result of a dynamic interaction between genes that 25 regulate the transition between vegetative and reproductive stages and those that regulate leaf 26 morphology and plant architecture. -
Domestication Syndrome” in Mammals: a Unified Explanation Based on Neural Crest Cell Behavior and Genetics
HIGHLIGHTED ARTICLE PERSPECTIVES The “Domestication Syndrome” in Mammals: A Unified Explanation Based on Neural Crest Cell Behavior and Genetics Adam S. Wilkins,*,†,1 Richard W. Wrangham,*,‡ and W. Tecumseh Fitch§ *Stellenbosch Institute of Advanced Study, Stellenbosch 7600, South Africa, †Institute of Theoretical Biology, Humboldt University zu Berlin, Berlin 10115, Germany, ‡Department of Human Evolutionary Biology, Harvard University, Cambridge, Massachusetts 02138, and §Department of Cognitive Biology, University of Vienna, A-1090 Vienna, Austria ABSTRACT Charles Darwin, while trying to devise a general theory of heredity from the observations of animal and plant breeders, discovered that domesticated mammals possess a distinctive and unusual suite of heritable traits not seen in their wild progenitors. Some of these traits also appear in domesticated birds and fish. The origin of Darwin’s “domestication syndrome” has remained a conundrum for more than 140 years. Most explanations focus on particular traits, while neglecting others, or on the possible selective factors involved in domestication rather than the underlying developmental and genetic causes of these traits. Here, we propose that the domestication syndrome results predominantly from mild neural crest cell deficits during embryonic development. Most of the modified traits, both morphological and physiological, can be readily explained as direct consequences of such deficiencies, while other traits are explicable as indirect consequences. We first show how the hypothesis can account for the multiple, apparently unrelated traits of the syndrome and then explore its genetic dimensions and predictions, reviewing the available genetic evidence. The article concludes with a brief discussion of some genetic and developmental questions raised by the idea, along with specific predictions and experimental tests.