Liversidge Research Lecture No
Total Page:16
File Type:pdf, Size:1020Kb
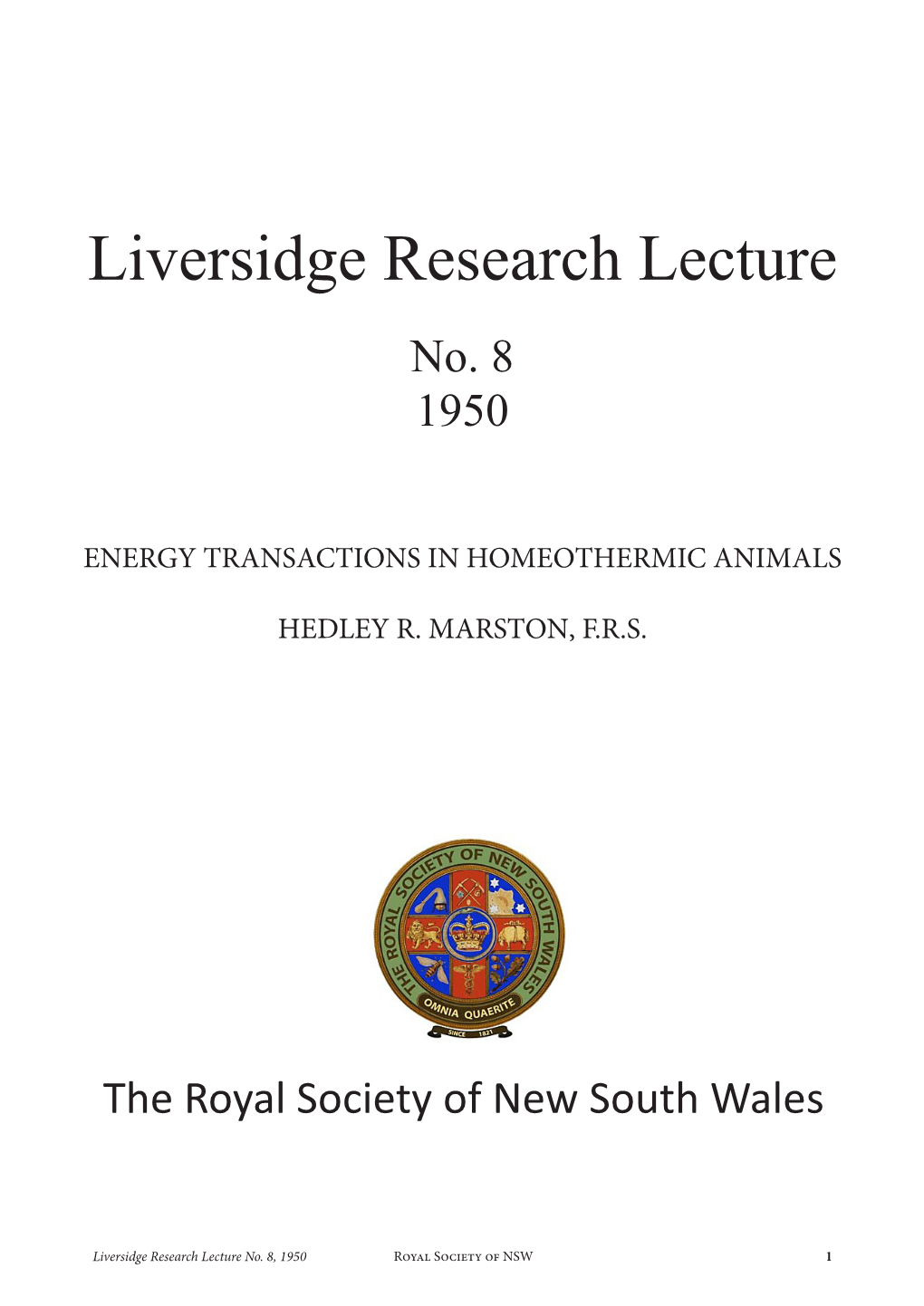
Load more
Recommended publications
-
Scientists' Houses in Canberra 1950–1970
EXPERIMENTS IN MODERN LIVING SCIENTISTS’ HOUSES IN CANBERRA 1950–1970 EXPERIMENTS IN MODERN LIVING SCIENTISTS’ HOUSES IN CANBERRA 1950–1970 MILTON CAMERON Published by ANU E Press The Australian National University Canberra ACT 0200, Australia Email: [email protected] This title is also available online at http://epress.anu.edu.au National Library of Australia Cataloguing-in-Publication entry Author: Cameron, Milton. Title: Experiments in modern living : scientists’ houses in Canberra, 1950 - 1970 / Milton Cameron. ISBN: 9781921862694 (pbk.) 9781921862700 (ebook) Notes: Includes bibliographical references and index. Subjects: Scientists--Homes and haunts--Australian Capital Territority--Canberra. Architecture, Modern Architecture--Australian Capital Territority--Canberra. Canberra (A.C.T.)--Buildings, structures, etc Dewey Number: 720.99471 All rights reserved. No part of this publication may be reproduced, stored in a retrieval system or transmitted in any form or by any means, electronic, mechanical, photocopying or otherwise, without the prior permission of the publisher. Cover design by Sarah Evans. Front cover photograph of Fenner House by Ben Wrigley, 2012. Printed by Griffin Press This edition © 2012 ANU E Press; revised August 2012 Contents Acknowledgments . vii Illustrations . xi Abbreviations . xv Introduction: Domestic Voyeurism . 1 1. Age of the Masters: Establishing a scientific and intellectual community in Canberra, 1946–1968 . 7 2 . Paradigm Shift: Boyd and the Fenner House . 43 3 . Promoting the New Paradigm: Seidler and the Zwar House . 77 4 . Form Follows Formula: Grounds, Boyd and the Philip House . 101 5 . Where Science Meets Art: Bischoff and the Gascoigne House . 131 6 . The Origins of Form: Grounds, Bischoff and the Frankel House . 161 Afterword: Before and After Science . -
Adelaidean Vol 6 No 12 14 July 1997
Adelaidean Vol 6 No 12 AdelaideanN EWS FROM THE UNIVERSITY OF ADELAIDE JULY 14, 1997 ‘Horse course’ targets industry needs The ‘horse course’ at the University of interested and willing to do whatever Adelaide’s Roseworthy Campus is it takes to get the work done. now well on track to produce gradu- “We want them to adopt a work ates who are better suited to the ethic so that when they get out into needs of industry. the real world they have suitable expe- The Diploma in Horse Husbandry rience and initiative, making them of and Management has this year under- value to their employer.” gone major changes following consul- Graduates of the course have a wide tation with representatives of the rac- range of career options open to them ing, training, riding and retail in the horse industries. This includes industries. working in stud management, racing, The two-year diploma course pro- horse-related recreational and service vides students with skills in the han- industries, nutrition, equine journal- dling, riding and training of horses, as ism, business, marketing and advertis- well as property, personnel and busi- ing. ness management. Occasionally one of the Roseworthy Students are responsible for main- Campus horses is bred for the racing taining the Roseworthy stables, and in industry. The current hopeful is a the second year of their course they yearling thoroughbred, the last foal undertake industry experience either bred at the campus. in Australia or overseas. “So far the second year students Mrs Annette Warendorf, who last have broken him in and done some year was the top graduate from the pre-training with him,” said Mrs Horse Husbandry and Management Warendorf. -
Genocide Perspectives VI Editors the Process and the Personal Cost of Genocide Marczak & Shields
Genocide Perspectives VI The Process and the Personal Cost of Genocide The Process and the Personal Cost of Genocide The Process Genocide Perspectives VI CONTRIBUTORS Alex J. Bellamy and Stephen Mark Tedeschi AM QC McLoughlin The 2017 Myall Creek Massacre Fateful Choices: Political Leadership and Commemoration Speech the Paths to and from Mass Atrocities Caroline Schneider and Hans-Lukas Melanie O’Brien Kieser Freedom of Religion in the Genocidal Long Shadows—The Great War, Process and Group Destruction in Australia and the Middle East: From the the Holocaust and Armenian and Armenian to the Yazidi Genocide Cambodian Genocides Armen Gakavian Katharine Gelber “It’s Happening Again”: Genocide, Denial, Post-memory and Artefacts: The Gelber/ Exile and Trauma Altschul Collection Amanda Tink “If You’re Different Are You the Same?”: The Nazi Genocide of Disabled People and Les Murray’s Fredy Neptune Linda Shields and Susan Benedict Nursing in Nazi Germany and the “Euthanasia” Programmes Marczak & Shields Marczak Colin Tatz Genocide and Suicide Editors Jacob G. Warren Apprehending the Slow Violence of Nuclear Colonialism: Art and Maralinga UTS EPRESS PUBLISHES PEER-REVIEWED, SCHOLARLY OPEN ACCESS BOOKS AND JOURNALS Genocide Perspectives VI The Process and the Personal Cost of Genocide Edited by Nikki Marczak and Kirril Shields Australian Institute for Holocaust and Genocide Studies Genocide Perspectives Series UTS ePRESS University of Technology Sydney Broadway NSW 2007 AUSTRALIA epress.lib.uts.edu.au Copyright Information This book is copyright. -
Animal Science Down Under: a History of Research, Development and Extension in Support of Australia’S Livestock Industries
CSIRO PUBLISHING Animal Production Science Reflections https://doi.org/10.1071/AN19161 Animal science Down Under: a history of research, development and extension in support of Australia’s livestock industries Alan W. Bell Department of Animal Science, Cornell University, Ithaca, NY 14853-4801, USA. Email: [email protected] Abstract. This account of the development and achievements of the animal sciences in Australia is prefaced by a brief history of the livestock industries from 1788 to the present. During the 19th century, progress in industry development was due more to the experience and ingenuity of producers than to the application of scientific principles; the end of the century also saw the establishment of departments of agriculture and agricultural colleges in all Australian colonies (later states). Between the two world wars, the Council for Scientific and Industrial Research was established, including well supported Divisions of Animal Nutrition and Animal Health, and there was significant growth in research and extension capability in the state departments. However, the research capacity of the recently established university Faculties of Agriculture and Veterinary Science was limited by lack of funding and opportunity to offer postgraduate research training. The three decades after 1945 were marked by strong political support for agricultural research, development and extension, visionary scientific leadership, and major growth in research institutions and achievements, partly driven by increased university funding and -
The Advertiser: Maralinga Dossier
The Advertiser: Maralinga Dossier A collection of articles by journalist Colin James. This collection used to be posted at: www.theadvertiser.news.com.au/sectionindex2/0%2C5935%2Cwoomera^woomera^T EXT%2C00.html ... but that page is no longer available and doesn't seem to be captured by web archive services (e.g. http://web.archive.org) List of articles in this file: Mums, babies had picnics during tests, 08 May 2003 www.theadvertiser.news.com.au/common/story_page/0,5936,6406995%255E26839,00.html Nuclear tests no one knew about, 10 June 2003, www.theadvertiser.news.com.au/common/story_page/0,5936,6571804%255E26839,00.html What killed the Woomera babies, 07 May 2003, www.theadvertiser.news.com.au/common/story_page/0,5936,6406997%255E26839,00.html Nuclear dust settled across 1950s Adelaide, 06 May 2003, www.theadvertiser.news.com.au/common/story_page/0,5936,6407001%255E26839,00.html Deaths, serious illness over three decades leads to call for inquiry, 03 May 2003 www.theadvertiser.news.com.au/common/story_page/0,5936,6407003%255E26839,00.html Just like your dad, 03 May 2003, www.theadvertiser.news.com.au/common/story_page/0,5936,6407060%255E26839,00.html Health card victory for test veterans, 08 Mar 2003, www.theadvertiser.news.com.au/common/story_page/0,5936,6407061%255E26839,00.html Shut your eyes and dig, British military's advice for surviving an A-bomb, 08 Jun 2002, www.theadvertiser.news.com.au/common/story_page/0,5936,6407063%255E26839,00.html Found: hidden documents on A-bomb tests, 24 April 2002, www.theadvertiser.news.com.au/common/story_page/0,5936,6407066%255E26839,00.html -
The Scientific Temper: an Anthology of Stories on Matters of Science By
Trav.els 1982-Canberra, the Science City * . L ~ ‘ Tit.le 316 I met two important scientists in Canberra during that visit whom I had not known before: Professor Ted Ringwood, the inventor of ‘Synrock’ and Dr Susan Bam- brick, Senior Lecturer in Economics, both of the Australian National University, ANU. Ringwood had the simple, but brilliant idea that highly radioactive waste products should be enclosed in his invention, synthetic rock. The waste would then be sufficiently inert to be deposited safely for a very long time, not unlike the ura- nium isotopes which had been preserved over a period of 1800 million years in the natural Oklo reactor in Gabon. [See Title 2631. I subsequently never heard of any large scale use of synrock, nor did I hear any arguments or read,of any reasons why synrock was unsuitable for the purpose for which it was invented. - . , I, Dr Bambrick was, at the time I met her, an expert in the economics of Australian mineral products and their exports, and I invited her to contribute her views about her field of research. She .wrote the article during8the following year when she was a Fulbright Scholar in residence at Pennsylvania State College; and it was published in September 1984, ISR 9/3. Lconsidered it an excellent interdisciplinary contribu- tion, ,dealing with economics, politics, export controls and environmental protec- tion. 8 , I. -+ . , Canberra has always been .a great attraction for. me, being *Australia’sFederal Capital and ‘Science City’. Apart from housing the Premier Research University of Australia, ANU, it also has the hemispherical home of the Australian National Academy, whose Fellows proudly place FAA behind their name, equivalent to FRS in the scientific hierarchy. -
Coresearch (1974)
176##1974 Produced by the Central Communication Unit for circulation among members of CSI RO staff January 1974 (SI RO to review its medical research policy More emphasis may be placed on nutrition CSIRO is cnrrently giv Professor Fenner H Iso sug 'This research is sometimes • influenza virus vaccine; syner~ • muscular dystrophies - Food gested that the Australian not satisfactorily exploited in gism of antihacterial com~ Research, Protein Chemistry, ing consideration to the im Government should: relalion to human health be pact of its work relating to pounds (working together o[ Nutritional Biochemistry • set lip an Australian Medical cause of the absence of effec antibacterial compounds with • collagens and ageing; woo] medical research and the Research Council separate tive liaison between CSIRO body (issues and substances) allergenic properties - Pro Executive proposes to create from the National Health and medical research workers, -Animal Genetics tein Chemistry and Medical Research Coun or because an organisation for e reprC?ductive physiology, en· • advice on statistical aspects a CSIRO Medical Research conducting tbe appropriate foJ-' Liaison Committee on cil, which would become the docnnolagy, polyunsaturated of many programmes of National Health Council low-up medical research does fat mCcHs and milk procJucts medical research - Mathe which both CSIRO and • establish an Australian not exist in Australia,' he said. - Animal Physiology, Food matical Statistics medical interests will be National Institute for Medi Informal -
Establishing a Scientific and Intellectual Community in Canberra, 1946–1968
1. Age of the Masters: Establishing a scientific and intellectual community in Canberra, 1946–1968 The Formation of The Australian National University Having proven their worth in wartime, scientists in the 1950s were as powerful as they ever had been, confident of their own capacity to change the world, and dismissive of those who stood in their way. — Stephen Foster and Margaret Varghese1 The architect must be entirely subordinated to the scientific requirements of those who are to inhabit [the building]…I will not be pushed around by an architect for architectural reasons. — Sir Howard Florey2 One evening in April 1946, Australian Prime Minister, Ben Chifley, in London for the first postwar Commonwealth Prime Ministers’ Conference, booked a large table for dinner at The Savoy Hotel in The Strand. Also dining with him that night was a thirty-six-year-old economist and planner, Herbert Cole (‘Nugget’) Coombs,3 whom he had appointed Director-General of the Commonwealth Department of Post-War Reconstruction, Dr Herbert Vere (‘Doc’) Evatt, the Minister for External Affairs, and other members of the official party. The Savoy, one of London’s most distinguished and elegant hotels, was upgrading its menu now that rationing was over, and under the French maître-chef, dishes from the Normandy region such as Tripes á la Mode de Caen and Canard á la Rouennaise were available. It is possible that former British Prime Minister Sir Winston Churchill was also there; a frequent patron, he was known to have dined there as often as five times a fortnight.4 But Chifley was not interested in the guests 1 Stephen G. -
Mark Oliphant and the Invisible College of the Peaceful Atom
The University of Notre Dame Australia ResearchOnline@ND Theses 2019 Mark Oliphant and the Invisible College of the Peaceful Atom Darren Holden The University of Notre Dame Australia Follow this and additional works at: https://researchonline.nd.edu.au/theses Part of the Arts and Humanities Commons COMMONWEALTH OF AUSTRALIA Copyright Regulations 1969 WARNING The material in this communication may be subject to copyright under the Act. Any further copying or communication of this material by you may be the subject of copyright protection under the Act. Do not remove this notice. Publication Details Holden, D. (2019). Mark Oliphant and the Invisible College of the Peaceful Atom (Doctor of Philosophy (College of Arts and Science)). University of Notre Dame Australia. https://researchonline.nd.edu.au/theses/270 This dissertation/thesis is brought to you by ResearchOnline@ND. It has been accepted for inclusion in Theses by an authorized administrator of ResearchOnline@ND. For more information, please contact [email protected]. Frontispiece: British Group associated with the Manhattan Project (Mark Oliphant Group), Nuclear Physics Research Laboratory, University of Liverpool, Mount Pleasant, Liverpool. 1944. Photograph by Donald Cooksey. General Records of the Department of Energy (record group 434). National Archives (US) Arc Identifier 7665196. https://catalog.archives.gov/id/7665196 Reproduced for non-commercial purposes. Copyright retained by The University of California. DOCTOR OF PHILOSOPHY Mark Oliphant And the Invisible College of the Peaceful Atom A thesis submitted in the fulfilment of a Doctor of Philosophy Darren Holden The University of Notre Dame Australia Submitted February 2019, revised June 2019 ii ACKNOWLEDGEMENTS As this work has attempted to demonstrate: discovery is not possible in isolation. -
Twentieth Century Heritage Survey Stage 1: Post Second World War (1946 -1959)
2088.04 Heritage surve#8CF8.qxd 6/1/05 10:42 AM Page 1 Department for Environment and Heritage Twentieth Century Heritage Survey Stage 1: Post Second World War (1946 -1959) Overview History Susan Marsden (Marsden Russell Historians) [assisted by Carol Cosgrove and Robyn Taylor] Adelaide 2003-4 www.environment.sa.gov.au 2088.04 Heritage surve#8CF8.qxd 6/1/05 10:42 AM Page 2 Front Cover: South Australians in the 1950s. The children are (left to right): Anthonius (Tony), Maria, Anthonia (Toosjie) and Johanna (Joh) in the summer of 1954/55. The family migrated from Holland in 1953 and have moved from migrant camps at Bonegilla and Finsbury to an asbestos house rented from the South Australian Housing Trust. Their father Anthonius Dam has photographed the four children in their front yard at 23 East Avenue, Northfield, enjoying a cold Coca- Cola, still a rare American treat for any South Australian child. Published by the Department for Environment and Heritage, Adelaide, South Australia For futher information contact: Heritage Branch Ground Floor 1 Richmond Road Keswick SA 5035 Ph: (61 8) 8124 4960 © Department for Environment, and Heritage, 2004 Revised January 2005, FIS 2088.04 Contents Introduction .................................................................................................................................5 Aims ...........................................................................................................................................5 Methodology...........................................................................................................................5 -
Trace Element Disorders in South Australian Agriculture
Trace Element disorders in South Australian Agriculture The successful development of modern agricultural industries in most regions of South Australia could not have been achieved without the discovery and correction of trace element deficiencies in crops, pastures, horticultural tree and vegetable crops, plantation forests and livestock. Over the past eight decades, the low trace element status of many SA agricultural soils has been demonstrated repeatedly and often multiple deficiencies were identified. Their amelioration with trace element fertilisers (together with macronutrients, phosphorus (P), nitrogen (N), potassium (K) and/or sulphur (S)) led to: • the development of large tracts of infertile land for agricultural production; • spectacular increases in yields and the quality of harvested products; and • marked improvements in livestock productivity, carrying capacity and the quality of livestock products. The research which underpinned these remarkable gains in agricultural productivity often paralleled or preceded similar research undertaken elsewhere in Australia, notably in Western Australia. In some cases, the advances had international application, such as establishing the cause for and effective measures for preventing “coast disease” in sheep. It is also worth noting that the pioneering researchers had to confront considerable scientific challenges, since they lacked the modern analytical technologies that we take for granted today. As Professors CM Donald and JA Prescott stated in 1975 at the Waite Institute Jubilee International Symposium on Trace Elements: “No country has derived greater benefit from trace elements than Australia”. Pioneering discoveries (1928 – 1960): In 1928, Dr CS Piper (a chemist) and Geoffrey Samuel (a plant pathologist) from the Waite Institute reported the first trace element disorder identified in Australia - manganese (Mn) deficiency in oats grown adjacent to limestone roads (known locally as ‘roadside takeall’ or ‘grey speck disease’) at Penola and Mount Gambier. -
Historical Records Australian Science
Publishing Historical Records of Australian Science Volume 14, 2002–03 © Australian Academy of Science 2002 All enquiries and manuscripts should be directed to: Professor R. W. Home Department of History and Philosophy of Science The University of Melbourne, Parkville, Vic. 3010, Australia Telephone: +61 3 8344 6556 Fax: +61 3 8344 7959 Email: [email protected] Published by CSIRO PUBLISHING for the Australian Academy of Science www.publish.csiro.au/journals/hras Historical Records of Australian Science, 2002, 14, 209–249 Book Review Section Compiled by Libby Robin with assistance from John Jenkin Centre for Resource and Environmental Studies (CRES), Australian National University, Canberra, ACT, 0200, Australia. Email: [email protected] Roger Cross: Fallout: Hedley Marston detachment and adds to our knowledge of and the British Bomb Tests in Australia. the politics and personalities involved in Wakefield Press: Kent Town, 2001. Australia’s atomic adventures. xii + 226 pp., illus., ISBN: 1 862 54523 5 Hedley Marston, Chief of CSIRO’s (PB), $24.95. Division of Biochemistry and General Nutrition, was approached by British authorities in 1955 to assist in studying the effects of radiation on animals. After initial firings in 1952–3, the British atomic testing program was about to recommence in the Monte Bello Islands, and at the newly-established mainland test site known as Maralinga. Marston decided to examine the take-up of radioactive fallout in grazing animals by measuring the concentration of radioactive iodine in their thyroid glands. His research indicated that fallout was being deposited over a much wider area than the physicists on the Atomic Weapons Test Safety Committee (AWTSC) had publicly admitted.