Micro Vs MEGA: Trends Influencing the Development of the Power System
Total Page:16
File Type:pdf, Size:1020Kb
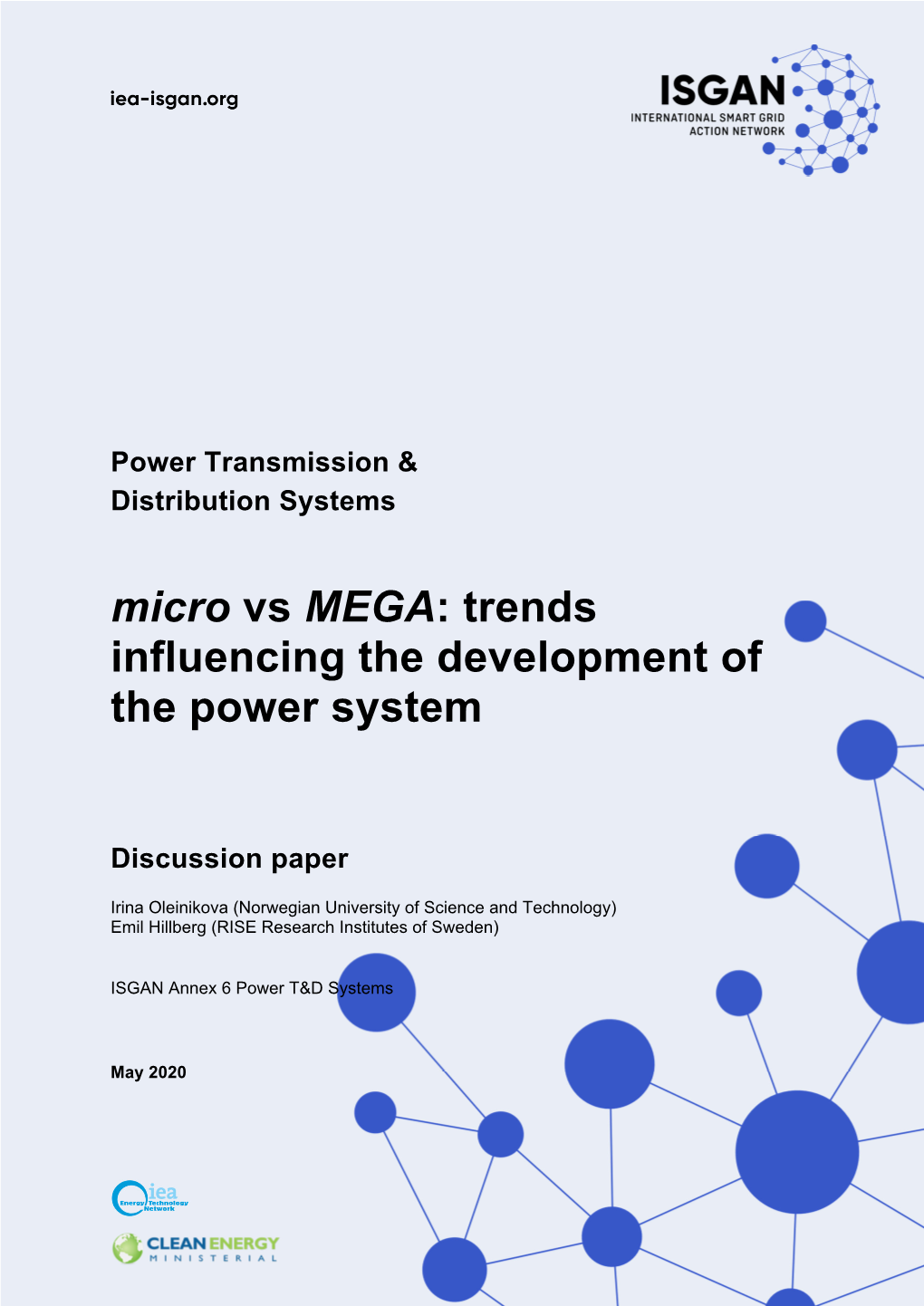
Load more
Recommended publications
-
Coal:25.0 Coal:31.0 :28.6 Just Before the After the Earthquake Earthquake FY 2010 FY 2014 Oil:6.6 Dependence Dependence Degree 62% Degree 88%
Magnesia Market Position Table of Contents Magnesia Division Yusuke Saitou FGD Market so far and from now on ①Recent trend in the domestic FGD market in Japan ②Chinese CCM supply situation to FGD market in Japan, Korea and Taiwan ③Movement of FGD facilities coming up in China Trend of Power Supply Composition in Japan Re-energy etc.:1.1 Re-energy etc.:3.2 Hydraulic power:8.5 Hydraulic power:9.0 Other gas: 1.1 Nuclear power Coal:25.0 Coal:31.0 :28.6 Just Before the After the Earthquake Earthquake FY 2010 FY 2014 Oil:6.6 Dependence Dependence degree 62% degree 88% Oil:9.5 Other gas:0.9 LNG :46.1 LNG :29.3 Source: Agency for Natural Resources and Energy ・After the earthquake, the proportion of coal-fired power has been increasing due to problems of nuclear power plant operation. Besides, LNG's proportion is also increasing. ・Domestic and Chinese MgO for FGD in part of coal-fired power generation (small & medium- sized )plants is around 10% share of total power generation. UBE MgO/MH Shipment ☆Compared to FY 2015, MH slurry shipment is expected to increase in FY 2020. ☆The anticipated increase in the quantity is expected to peak until 2020. 1,000mt(as MgO) UBE Material Estimated COP21 CO2 Regulation in the Paris Agreement 【Paris Agreement】 ・ We aim to make the global temperature rise of the whole world to 2℃ or lower, or even 1.5 ℃ or lower ・ Each country strengthens regulations, such as reporting CO2 missions every five years Source: draft committee submitted to the United Nations Framework Convention on Climate Change Asian FGD Market and Chinese CCM Trend Liaoning Province, Xiuyan District ( high purity CCM for FGD & large crystal FM ) Chinese CCM(30%) China 20,000? Unit: Mt / year (estimate as MgO) Domestic(70%) Korea 50,000 Japan Taiwan 200,000 China Expand? Japan Export 50,000 Chinese CCM (100%) North Korea With the environmental inspections by the central gov. -
The Asian Super Grid アジアの巨大配電網
Volume 10 | Issue 48 | Number 1 | Article ID 3858 | Jun 29, 2016 The Asia-Pacific Journal | Japan Focus The Asian Super Grid アジアの巨大配電網 The integration of East Asia is a topic of Newcom has already established a track perennial interest – whether it be monetary record, building Mongolia’s first wind farm in integration (much discussed in the wake of the record time, and bringing it to fruition by the 1997 financial crisis), trade integrationend of 2012. Mongolia is a classic instance of a (promoted via ever-expanding FTA areas) or latecomer country powering ahead through even political integration. But what is not heavy utilization of its vast fossil fuel reserves widely discussed (as yet) is actually the best (mainly coal). It is a country sharing major hope for effective integration – and that is borders with China and Russia that has had energy integration, via an Asian super grid unprecedented flows of inward foreign linking the enhanced electric power systems of investment from companies like Rio Tinto to China, Japan, Korea, Mongolia and perhaps build its coal export industry – much of that Russia. coal going to power China’s black industrial revolution. But at the same time, Mongolia is Just such an Asian Super Grid has been seeing the development of vast wind farms that proposed – by the charismatic Softbank CEO promise a genuine green revolution. Newcom, Son Masayoshi, driver of Japan’s post-led by its English-speaking CEO, Bayanjargal Fukushima shift to a renewable energyByambasaikhan, has created a huge new wind pathway. The first steps towards the Asian farm at Salkhit, just outside the capital Ulan Super Grid (ASG) were taken in October, when Bator, where 31 wind turbines are being SB Renewables, Son’s new subsidiarybrought into commission to generate 5% of the specializing in renewable energy, announced country’s power needs (now totally dependent an agreement with a company in Mongolia, on coal). -
Regional Power Grid Connectivity for Sustainable Development in North-East Asia
Regional Power Grid Connectivity for Sustainable Development in North-East Asia Policies and Strategies Note: The shaded areas of the map indicate ESCAP members and associate members.* The Economic and Social Commission for Asia and the Pacific (ESCAP) serves as the United Nations’ regional hub promoting cooperation among countries to achieve inclusive and sustainable development. The largest regional intergovernmental platform with 53 Member States and 9 Associate Members, ESCAP has emerged as a strong regional think- tank offering countries sound analytical products that shed insight into the evolving economic, social and environmental dynamics of the region. The Commission’s strategic focus is to deliver on the 2030 Agenda for Sustainable Development, which it does by reinforcing and deepening regional cooperation and integration to advance connectivity, financial cooperation and market integration. ESCAP’s research and analysis coupled with its policy advisory services, capacity building and technical assistance to governments aims to support countries’ sustainable and inclusive development ambitions. Note: *The designations employed and the presentation of material on this map do not imply the expression of any opinion whatsoever on the part of the Secretariat of the United Nations concerning the legal status of any country, territory, city or area or of its authorities, or concerning the delimitation of its frontiers or boundaries. Regional Power Grid Connectivity for Sustainable Development in North-East Asia Policies and Strategies United Nations publication Copyright © United Nations 2020 All rights reserved ST/ESCAP/2920 This publication may be reproduced in whole or in part for educational or nonprofit purposes without special permission from the copyright holder, provided that the source is acknowledged. -
Technical Assistance Consultant's Report Mongolia: Strategy For
Technical Assistance Consultant’s R eport Project Number: 48030-001 February 2020 Mongolia: S trategy for Northeast Asia Power S ystem Interconnection (Cofinanced by the Climate Change Fund, the People’s R epublic of China R egional Cooperation and Poverty R eduction Fund, and the R epublic of Korea e-Asia and Knowledge Partnership Fund) Prepared by E lectricite de France Paris, France For the Ministry of E nergy, Mongolia This consultant’s report does not necessarily reflect the views of ADB or the Government concerned, and ADB and the Government cannot be held liable for its contents. TA 9001-MON: Strategy for Northeast Asia Power S ystem Interconnections EDF References: CIST – DCO – PhL – 17 - 228 This consultant’s report does not necessarily reflect the views of ADB or the Government concerned, and ADB and the Government cannot be held liable for its contents. Strategy for NAPSI Inception Report - June 2017 FOREWORD The project Team would like to thank: - The Ministry of Energy of Mongolia for easing direct discussions with the National Dispatching Center, TRANSCO and Public Entities in Mongolia - TСe ADB’s Country CoordТnators of MonРolТa, People’s RepublТc of CСТna, RepublТc of Korea, Japan for their support: Mongolia: Mr. Byambasaikhan PRC: Ms. Geng Dan (Danna) ROK: Mr. Jung-Hwan Kim Japan: Mr. Omatsu Ryo and Mr. Shota Ichimura Here is a reminder of the place of the Module 1 in the Project organization: 1 EDF ELECTRICITE DE FRANCE – with a capital of 1 006 625 696.50 euros – TA-9001 MON: Strategy for Northeast Asia Power System Interconnection 552 081 371 R.C.S. -
Exploring Sociotechnical Pathways Towards Future Electricity Systems
$## "$" $ " ! # !) "#$ & 2=2:2767/6=29765.6;*4#@:;.5:6*4@:2: .8*9;5.6;7/$.,167470@*6*0.5.6;*6-,76752,: 5.902606.;>793:7/87>.9 ?8479260 :7,27;.,162,*4 8*;1>*@: ;7>*9-: /<;<9. .4.,;92,2;@ :@:;.5: +*:.- 76 9.6.>*+4. .6.90@;.,1674702.: "#$ & # B"#$ & 73;79:*=1*6-4260*9=2-1*45.9:;.362:3*1A0:374* @:.92.69 ## ( .8*9;5.6;7/$.,167470@*6*0.5.6;*6-,76752,: 1*45.9:%62=.9:2;@7/$.,167470@ # 7;1.6+<90 #>.-.6 $.4.8176. 1*45.9:-202;*4;9@,3 7;1.6+<90#>.-.6 '2442*54*3. &*!#'!',/(*$+( )(/* 0)%(*#'!+(#(,"'#%),"/1+,(/*+ -,-*%,*##,1+1+,&++('*'/%'*!1,"'(%(!#+ :@41122;>@@;2534@/859-@1/4-:31181/@>5/5@E?E?@19?->;A:0@41C;>80->1A:01>3;5:3- @>-:?5@5;: 2>;9 2;??582A18810 @; >1:1C-.81 1:1>3E.-?10 <>;0A/@5;: ;2 181/@>5/5@E '41 @>-:?5@5;:/-:4;C1B1>.1-@@-5:105:?1B1>-8>-05/-88E05221>1:@C-E?>-:35:32>;938;.-8;> /;:@5:1:@-8 ?A<1>3>50? B5- 8;/-8 ?9->@3>50? @; ?182?A225/51:@ ;223>50 /;99A:5@51? -:0 4;A?14;80? ;2 181/@>5/5@E <>;?A91>? ;C @41 @>-:?5@5;: C588 A:2;80 -:0 C4-@ @41 1B1:@A-8 ?E?@19C5888;;78571>19-5:?A:/1>@-5:E?452@5:32;/A?-C-E2>;9?59<8E5:/>1-?5:3@41 ?4->1;2>1:1C-.811:1>3E<>;0A/@5;:@;@41?<1/525//;:253A>-@5;:?;2>1:1C-.81?C1/-: .1@@1>:-B53-@1@41/;9<81D@1/4:;8;35/-88-:0?/-<1.1@@1>@->31@5:B1?@91:@-:0418<5:2;>9 @413;B1>:91:@?.A?5:1??1?-:0/5@5F1:?@4-@->1?4-<5:3@412A@A>1;2@41181/@>5/5@E?E?@19 '45?@41?5?-59?@;?@A0E@41191>31:/1;2-8@1>:-@5B1181/@>5/5@E?E?@19/;:253A>-@5;:?@4-@ /;A80 ?-@5?2E @41 />5@1>5;: -
Smart Power Grids and Integration of Renewables in Japan
Background Study Smart power grids and integration of renewables in Japan Status and activities concerning smart grids implementation and integration of renewable energy sources in Japan Miha Jensterle (adelphi), Maike Venjakob (Wuppertal Institute) adelphi Wuppertal Institute I This study was compiled in the frame of the project "Supporting the Energy Dialogue with Japan and Supporting the Bilateral Energy Relations with Korea" on behalf of the Federal Office of Economic Affairs and Export Control (BAFA) and was prepared on request of Division IIA2 of the Federal Ministry for Economic Affairs and Energy (BMWi). The authors would like to thank Ms. Sichao Kan from the IEEJ and Mr. Masayuki Dewaki from NEDO for their contributions. Suggested Citation Jensterle, Miha; Maike Venjakob 2019: Smart power grids and integration of renewables in Japan. Current activities concerning smart grids implementation, energy system digitisation and integration of renewables. Berlin: adelphi. Imprint Publisher: adelphi consult GmbH Alt-Moabit 91 10559 Berlin +49 (030) 8900068-0 [email protected] www.adelphi.de Authors: Miha Jensterle (adelphi), Maike Venjakob (Wuppertal Institute) Layout: adelphi Photo credits: think4photop - shutterstock.com Status: 17.10.2019 © 2019 adelphi II adelphi Wuppertal Institute Executive Summary Like many other countries depending heavily on energy imports, Japan is trying to reduce its vulnerability to price shocks and geopolitical risks. It also set itself the goal of substantially reducing its greenhouse gas emissions by mid-century, and needs to keep the energy prices at affordable levels. This classic energy trilemma is flanked in Japan by the pressing need for improving the safety issues relating to energy supply, exposed by the environmental catastrophe and massive blackouts brought about by the Fukushima Daiichi disaster in 2011. -
Renewable Potential in Gobi Desert, Gobitec and Asian Super Grid Initiative
The 4th Northeast Asia Energy Security Forum 2016 Seoul, December 15 2016 Renewable potential in Gobi desert, Gobitec and Asian Super Grid Initiative Yeren-Ulzii Batmunkh, Senior Officer, Ministry of Energy, Mongolia Contents Renewable Potential in Gobi Desert Wind, Solar Gobitec Initiative Idea, Concept Towards Asian Super Grid Rationale, What is next Concluding Lessons to be learned, recommendations www.energy.gov.mn www.energy.gov.mn www.energy.gov.mn Solar – Solar: 270-300 sunny days in a year, 4.3-4.7 kWh/meter or higher per day Estimated kWh/ m2/day Applicable land (km2) Total Power: GWh/year 3.4 5,269.5 654,000 3.8 3,924.7 544,000 4.1 4,210.6 630,000 4.5 4,514.8 742,000 5.4 5,541.9 1,092,000 Total 23,461.5 4,774,000 www.energy.gov.mn •Source: US National Renewable Energy Laboratory, National Renewable Energy Center of Mongolia Renewable Potential in Gobi Desert National Renewable Energy Laboratory and the US Department of Energy estimates the overall potential for installed renewable energy by 2,600 TWh, only for the Mongolian part of the Gobi desert. This divides into 1,100 TWh wind energy potential and 1,500 TWh solar power potential per year. Therefore after an estimation, 3% of the Gobi area would be enough, which includes around 1,3 Mio. km² to produce more than 21.400 TWh of energy in one year. Calculations existed, that this energy production per year would have been enough to serve the energy demand of the whole world in the year 2008.