LKB1 Negatively Regulates AKT1 Signaling Via DBC1 and TRB3
Total Page:16
File Type:pdf, Size:1020Kb
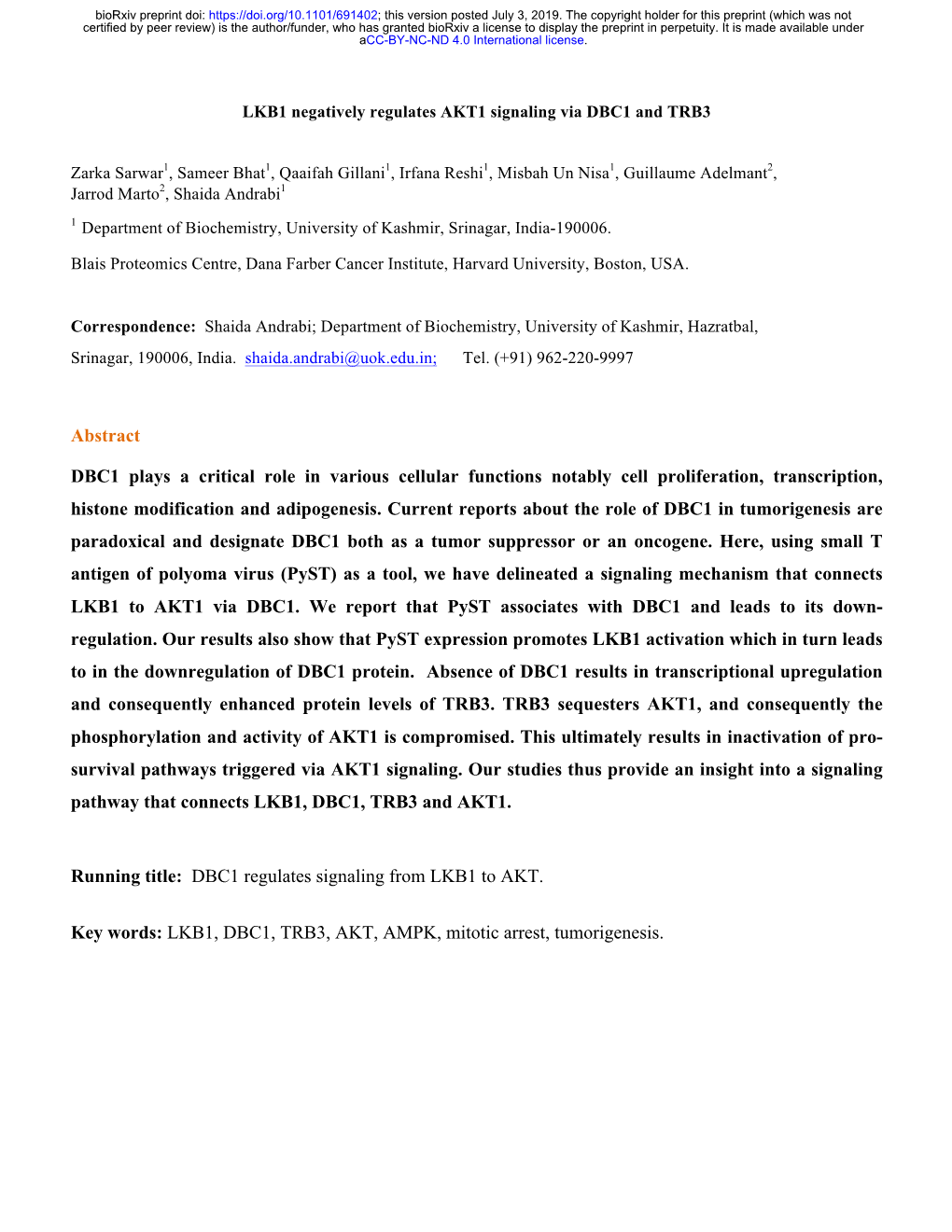
Load more
Recommended publications
-
Aneuploidy: Using Genetic Instability to Preserve a Haploid Genome?
Health Science Campus FINAL APPROVAL OF DISSERTATION Doctor of Philosophy in Biomedical Science (Cancer Biology) Aneuploidy: Using genetic instability to preserve a haploid genome? Submitted by: Ramona Ramdath In partial fulfillment of the requirements for the degree of Doctor of Philosophy in Biomedical Science Examination Committee Signature/Date Major Advisor: David Allison, M.D., Ph.D. Academic James Trempe, Ph.D. Advisory Committee: David Giovanucci, Ph.D. Randall Ruch, Ph.D. Ronald Mellgren, Ph.D. Senior Associate Dean College of Graduate Studies Michael S. Bisesi, Ph.D. Date of Defense: April 10, 2009 Aneuploidy: Using genetic instability to preserve a haploid genome? Ramona Ramdath University of Toledo, Health Science Campus 2009 Dedication I dedicate this dissertation to my grandfather who died of lung cancer two years ago, but who always instilled in us the value and importance of education. And to my mom and sister, both of whom have been pillars of support and stimulating conversations. To my sister, Rehanna, especially- I hope this inspires you to achieve all that you want to in life, academically and otherwise. ii Acknowledgements As we go through these academic journeys, there are so many along the way that make an impact not only on our work, but on our lives as well, and I would like to say a heartfelt thank you to all of those people: My Committee members- Dr. James Trempe, Dr. David Giovanucchi, Dr. Ronald Mellgren and Dr. Randall Ruch for their guidance, suggestions, support and confidence in me. My major advisor- Dr. David Allison, for his constructive criticism and positive reinforcement. -
Understanding the Role of Perilipin 5 in Non-Alcoholic Fatty Liver Disease and Its Role in Hepatocellular Carcinoma: a Review of Novel Insights
International Journal of Molecular Sciences Review Understanding the Role of Perilipin 5 in Non-Alcoholic Fatty Liver Disease and Its Role in Hepatocellular Carcinoma: A Review of Novel Insights Paola Berenice Mass Sanchez, Marinela Krizanac, Ralf Weiskirchen * and Anastasia Asimakopoulos * Institute of Molecular Pathobiochemistry, Experimental Gene Therapy and Clinical Chemistry (IFMPEGKC), RWTH University Hospital Aachen, D-52074 Aachen, Germany; [email protected] (P.B.M.S.); [email protected] (M.K.) * Correspondence: [email protected] (R.W.); [email protected] (A.A.) Abstract: Consumption of high-calorie foods, such as diets rich in fats, is an important factor leading to the development of steatohepatitis. Several studies have suggested how lipid accumulation creates a lipotoxic microenvironment for cells, leading cells to deregulate their transcriptional and transla- tional activity. This deregulation induces the development of liver diseases such as non-alcoholic fatty liver disease (NAFLD) and subsequently also the appearance of hepatocellular carcinoma (HCC) which is one of the deadliest types of cancers worldwide. Understanding its pathology and studying new biomarkers with better specificity in predicting disease prognosis can help in the personalized treatment of the disease. In this setting, understanding the link between NAFLD and HCC progression, the differentiation of each stage in between as well as the mechanisms underlying this process, are vital for development of new treatments and in exploring new therapeutic targets. Citation: Mass Sanchez, P.B.; Perilipins are a family of five closely related proteins expressed on the surface of lipid droplets (LD) in Krizanac, M.; Weiskirchen, R.; several tissues acting in several pathways involved in lipid metabolism. -
Identification of 5 Novel Genes Methylated in Breast and Other Epithelial Cancers
Hill et al. Molecular Cancer 2010, 9:51 http://www.molecular-cancer.com/content/9/1/51 RESEARCH Open Access Identification of 5 novel genes methylated in breast and other epithelial cancers Victoria K Hill1, Luke B Hesson1,2, Temuujin Dansranjavin3, Ashraf Dallol1, Ivan Bieche4, Sophie Vacher4, Stella Tommasi5, Timothy Dobbins2, Dean Gentle1, David Euhus6, Cheryl Lewis6, Reinhard Dammann3, Robyn L Ward2, John Minna7, Eammon R Maher1, Gerd P Pfeifer5, Farida Latif1* Abstract Background: There are several high throughput approaches to identify methylated genes in cancer. We utilized one such recently developed approach, MIRA (methylated-CpG island recovery assay) combined with CpG island arrays to identify novel genes that are epigenetically inactivated in breast cancer. Results: Using this approach we identified numerous CpG islands that demonstrated aberrant DNA methylation in breast cancer cell lines. Using a combination of COBRA and sequencing of bisulphite modified DNA, we confirmed 5 novel genes frequently methylated in breast tumours; EMILIN2, SALL1, DBC1, FBLN2 and CIDE-A. Methylation frequencies ranged from between 25% and 63% in primary breast tumours, whilst matched normal breast tissue DNA was either unmethylated or demonstrated a much lower frequency of methylation compared to malignant breast tissue DNA. Furthermore expression of the above 5 genes was shown to be restored following treatment with a demethylating agent in methylated breast cancer cell lines. We have expanded this analysis across three other common epithelial cancers (lung, colorectal, prostate). We demonstrate that the above genes show varying levels of methylation in these cancers. Lastly and most importantly methylation of EMILIN2 was associated with poorer clinical outcome in breast cancer and was strongly associated with estrogen receptor as well as progesterone receptor positive breast cancers. -
Transdifferentiation of Human Mesenchymal Stem Cells
Transdifferentiation of Human Mesenchymal Stem Cells Dissertation zur Erlangung des naturwissenschaftlichen Doktorgrades der Julius-Maximilians-Universität Würzburg vorgelegt von Tatjana Schilling aus San Miguel de Tucuman, Argentinien Würzburg, 2007 Eingereicht am: Mitglieder der Promotionskommission: Vorsitzender: Prof. Dr. Martin J. Müller Gutachter: PD Dr. Norbert Schütze Gutachter: Prof. Dr. Georg Krohne Tag des Promotionskolloquiums: Doktorurkunde ausgehändigt am: Hiermit erkläre ich ehrenwörtlich, dass ich die vorliegende Dissertation selbstständig angefertigt und keine anderen als die von mir angegebenen Hilfsmittel und Quellen verwendet habe. Des Weiteren erkläre ich, dass diese Arbeit weder in gleicher noch in ähnlicher Form in einem Prüfungsverfahren vorgelegen hat und ich noch keinen Promotionsversuch unternommen habe. Gerbrunn, 4. Mai 2007 Tatjana Schilling Table of contents i Table of contents 1 Summary ........................................................................................................................ 1 1.1 Summary.................................................................................................................... 1 1.2 Zusammenfassung..................................................................................................... 2 2 Introduction.................................................................................................................... 4 2.1 Osteoporosis and the fatty degeneration of the bone marrow..................................... 4 2.2 Adipose and bone -
DBC1 Antibody A
C 0 2 - t DBC1 Antibody a e r o t S Orders: 877-616-CELL (2355) [email protected] Support: 877-678-TECH (8324) 3 9 Web: [email protected] 6 www.cellsignal.com 5 # 3 Trask Lane Danvers Massachusetts 01923 USA For Research Use Only. Not For Use In Diagnostic Procedures. Applications: Reactivity: Sensitivity: MW (kDa): Source: UniProt ID: Entrez-Gene Id: WB, IP, IF-IC H M R Mk Endogenous 130 Rabbit Q8N163 57805 Product Usage Information 1. Hamaguchi, M. et al. (2002) Proc Natl Acad Sci USA 99, 13647-52. 2. Trauernicht, A.M. et al. (2007) Mol Endocrinol 21, 1526-36. Application Dilution 3. Zhao, W. et al. (2008) Nature 451, 587-90. 4. Li, Z. et al. (2009) J Biol Chem 284, 10361-6. Western Blotting 1:1000 5. Sundararajan, R. et al. (2005) Oncogene 24, 4908-20. Immunoprecipitation 1:50 Immunofluorescence (Immunocytochemistry) 1:100 Storage Supplied in 10 mM sodium HEPES (pH 7.5), 150 mM NaCl, 100 µg/ml BSA and 50% glycerol. Store at –20°C. Do not aliquot the antibody. Specificity / Sensitivity DBC1 Antibody detects endogenous levels of total DBC1 protein. Species Reactivity: Human, Mouse, Rat, Monkey Species predicted to react based on 100% sequence homology: Horse Source / Purification Polyclonal antibodies are produced by immunizing animals with a synthetic peptide corresponding to residues near the amino terminus of human DBC1 protein. Antibodies are purified by protein A and peptide affinity chromatography. Background Deleted in breast cancer gene 1 protein (DBC1) was originally identified by its localization to a region of chromosome 8p21 that is homozygously deleted in breast cancer (1). -
Dephosphorylation of DBC1 by Protein Phosphatase 4 Is Important for P53-Mediated Cellular Functions
Dephosphorylation of DBC1 by Protein Phosphatase 4 Is Important for p53-Mediated Cellular Functions The Harvard community has made this article openly available. Please share how this access benefits you. Your story matters Citation Lee, Jihye, Guillaume Adelmant, Jarrod A. Marto, and Dong-Hyun Lee. 2015. “Dephosphorylation of DBC1 by Protein Phosphatase 4 Is Important for p53-Mediated Cellular Functions.” Molecules and Cells 38 (8): 697-704. doi:10.14348/molcells.2015.0066. http:// dx.doi.org/10.14348/molcells.2015.0066. Published Version doi:10.14348/molcells.2015.0066 Citable link http://nrs.harvard.edu/urn-3:HUL.InstRepos:22857022 Terms of Use This article was downloaded from Harvard University’s DASH repository, and is made available under the terms and conditions applicable to Other Posted Material, as set forth at http:// nrs.harvard.edu/urn-3:HUL.InstRepos:dash.current.terms-of- use#LAA Mol. Cells 2015; 38(8): 697-704 http://dx.doi.org/10.14348/molcells.2015.0066 Molecules and Cells http://molcells.org Established in 1990 Dephosphorylation of DBC1 by Protein Phosphatase 4 Is Important for p53-Mediated Cellular Functions Jihye Lee, Guillaume Adelmant1, Jarrod A. Marto1, and Dong-Hyun Lee* Deleted in breast cancer-1 (DBC1) contributes to the regu- ATM/ATR kinases (Zannini et al., 2012) and it plays a critical lation of cell survival and apoptosis. Recent studies role in maintaining genomic stability and cellular integrity follow- demonstrated that DBC is phosphorylated at Thr454 by ing genotoxic stress (Kim et al., 2008; 2009a; Magni et al., 2014; ATM/ATR kinases in response to DNA damage, which is a Park et al., 2014; Zannini et al., 2012; Zheng et al., 2013). -
Identification of 5 Novel Genes Methylated in Breast and Other Epithelial Cancers
Identification of 5 novel genes methylated in breast and other epithelial cancers. Victoria Hill, Luke Hesson, Temuujin Dansranjavin, Ashraf Dallol, Ivan Bieche, Sophie Vacher, Stella Tommasi, Timothy Dobbins, Dean Gentle, David Euhus, et al. To cite this version: Victoria Hill, Luke Hesson, Temuujin Dansranjavin, Ashraf Dallol, Ivan Bieche, et al.. Identification of 5 novel genes methylated in breast and other epithelial cancers.. Molecular Cancer, BioMed Central, 2010, 9 (1), pp.51. 10.1186/1476-4598-9-51. inserm-00663769 HAL Id: inserm-00663769 https://www.hal.inserm.fr/inserm-00663769 Submitted on 27 Jan 2012 HAL is a multi-disciplinary open access L’archive ouverte pluridisciplinaire HAL, est archive for the deposit and dissemination of sci- destinée au dépôt et à la diffusion de documents entific research documents, whether they are pub- scientifiques de niveau recherche, publiés ou non, lished or not. The documents may come from émanant des établissements d’enseignement et de teaching and research institutions in France or recherche français ou étrangers, des laboratoires abroad, or from public or private research centers. publics ou privés. Hill et al. Molecular Cancer 2010, 9:51 http://www.molecular-cancer.com/content/9/1/51 RESEARCH Open Access Identification of 5 novel genes methylated in breast and other epithelial cancers Victoria K Hill1, Luke B Hesson1,2, Temuujin Dansranjavin3, Ashraf Dallol1, Ivan Bieche4, Sophie Vacher4, Stella Tommasi5, Timothy Dobbins2, Dean Gentle1, David Euhus6, Cheryl Lewis6, Reinhard Dammann3, Robyn L Ward2, John Minna7, Eammon R Maher1, Gerd P Pfeifer5, Farida Latif1* Abstract Background: There are several high throughput approaches to identify methylated genes in cancer. -
Regulate Cancer?
NIH Public Access Author Manuscript Cellscience. Author manuscript; available in PMC 2009 September 10. NIH-PA Author ManuscriptPublished NIH-PA Author Manuscript in final edited NIH-PA Author Manuscript form as: Cellscience. 2008 April 1; 4(4): 50±56. Can Sir(2) regulate cancer? Pratibha V. Nerurkar1 and Vivek R. Nerurkar2 1 Laboratory of Metabolic Disorders and Alternative Medicine, Dept. of Molecular Biosciences and Bioengineering (MBBE), CTAHR, , University of Hawaii, Honolulu 2 Retrovirology Research Laboratory, Dept. of Tropical Medicine, Medical Microbiology & Pharmacology, Asia-Pacific Institute of Tropical Medicine and Infectious Diseases, John A. Burns School of Medicine, University of Hawaii, Honolulu Abstract Sirtuin activators, including small molecules such as polyphenols and resveratrol, are much desired due to their potential to ameliorate metabolic disorder and delay or prevent aging. In contrast, recent studies demonstrate that targeted silencing of sirtuin 1 (SIRT1) expression or activity by the deleted in breast cancer 1 (DBC1) may be beneficial by promoting p53-induced apoptosis in cancer cells, and by sensitizing cancerous cells to radiation therapy. Negative SIRT1 regulation also alleviates gene-repression associated with fragile X mental retardation syndrome. The targeted activation or inhibition of SIRT1 activity therefore emerges as a critical point of regulation in disease pathogenesis. Sirtuin 1 (SIRT1), mammalian homologue of the silencing information regulator 2 (Sir2) yeast gene, is a class III nicotinamide adenine dinucleotide (NAD+)-dependent histone deacetylase that is conserved across species and plays an important role in extending lifespan [1]. Located within the nucleus, SIRT1 regulates cell survival by modulating cellular stress, metabolic pathways and apoptosis. Although the numerous targets and functions of SIRT1 are constantly being unraveled (as summarized in Figure 1 and reviewed in [2–6]), cell survival was initially demonstrated to involve the SIRT-associated deacetylation of tumor suppressor gene, p53 [7–9]. -
Negative Regulation of G1/S Transition by the Candidate Bladder Tumour Suppressor Gene DBCCR1
Oncogene (2001) 20, 2956 ± 2964 ã 2001 Nature Publishing Group All rights reserved 0950 ± 9232/01 $15.00 www.nature.com/onc Negative regulation of G1/S transition by the candidate bladder tumour suppressor gene DBCCR1 Hiroyuki Nishiyama1, Jason H Gill1, Eva Pitt1, Wendy Kennedy1 and Margaret A Knowles*,1 1ICRF Clinical Centre, St. James's University Hospital, Beckett Street, Leeds, LS9 7TF, UK Deletion of all or part of chromosome 9q is the most and/or 9q and one of these genes might represent a common genetic alteration in all stages and grades of `gatekeeper' for urothelial cells (Kinzler and Vogelstein, bladder cancer. DBCCR1 (deleted in bladder cancer 1996). Detailed LOH studies of chromosome 9q in chromosome region candidate 1) maps to the chromosome TCC have indicated that there are several candidate region 9q32-33, a candidate tumour suppressor locus for tumour suppressor loci (Cairns et al., 1993; Dalbagni et bladder cancer. Although no mutations of DBCCR1 have al., 1993; Olumi et al., 1990; Simoneau et al., 1999), been detected in bladder tumours, expression of DBCCR1 one of which is at 9q32-33 (Habuchi et al., 1995, 1997; is silenced by promoter hypermethylation in 50% of Nishiyama et al., 1999b). The putative tumour bladder cancer cell lines analysed. Here we sought to suppressor gene in this region has been designated provide functional evidence to authenticate DBCCR1 as a DBC1 (deleted in bladder cancer 1). Using YAC and tumour suppressor using gene-transfer methods. Exogen- PAC contigs spanning the DBC1 region, we identi®ed a ous expression of DBCCR1 protein or an HA epitope- candidate tumour suppressor gene, DBCCR1 (deleted tagged fusion protein, HA-DBCCR1 in NIH3T3 cells and in bladder cancer chromosomal region candidate 1) human bladder tumour cell lines resulted in suppression of (Habuchi et al., 1998; Nishiyama et al., 1999a). -
AM 112.Indd 3 04.05.12 11:31 the Genes Found to Be Associated with SZ Using Computer Ance of Researched Population, and Linkage Disequilibrium Gene Databases (28, 46)
REVIEW ARTICLE GENOME ‑WIDE ASSOCIATION STUDIES IN SCHIZOPHRENIA, AND POTENTIAL ETIOLOGICAL AND FUNCTIONAL IMPLICATIONS OF THEIR RESULTS Ladislav Hosák1,2, Petr Šilhan2, Jiřina Hosáková2 Charles University in Prague, Faculty of Medicine in Hradec Králové, and University Hospital Hradec Králové, Czech Repub‑ lic: Department of Psychiatry1; University of Ostrava, Faculty of Medicine, and University Hospital Ostrava, Czech Republic: Department of Clinical Studies and Department of Psychiatry2 Summary: Background: Despite the fact that the genetic basis of schizophrenia has been intensively studied for more than two decades, our contemporary knowledge in this field is rather fractional, and a substantial part of it is still missing. The aim of this review article is to sum up the data coming from genome ‑wide association genetic studies in schizophrenia, and indicate prospective directions of further scientific endeavour. Methods: We searched the National Human Genome Research Institute’s Catalog of genome‑wide association studies for schizophrenia to identify all papers related to this topic. In consequence, we looked up the possible relevancy of these findings for etiology and pathogenesis of schizophrenia using the computer gene and PubMed databases. Results: Eighteen genome‑wide association studies in schizophrenia have been published till now, referring to fifty‑seven genes supposedly involved into schizophrenia’s etiopathogenesis. Most of these genes are related to neurodevelopment, neuroendocrinology, and immunology. Conclusions: It -
The Proteomic Profile of Deleted in Breast Cancer 1 (DBC1) Interactions Points to a Multifaceted Regulation of Gene Expression*□S
crossmark Research © 2016 by The American Society for Biochemistry and Molecular Biology, Inc. This paper is available on line at http://www.mcponline.org The Proteomic Profile of Deleted in Breast Cancer 1 (DBC1) Interactions Points to a Multifaceted Regulation of Gene Expression*□S Sophie S. B. Gigue`re‡¶, Amanda J. Guise‡¶, Pierre M. Jean Beltran‡¶, Preeti M. Joshi‡¶, Todd M. Greco‡, Olivia L. Quach‡, Jeffery Kong‡, and Ileana M. Cristea‡§ Deleted in breast cancer 1 (DBC1) has emerged as an nance of the circadian molecular clock. Furthermore, the important regulator of multiple cellular processes, rang- identified interactions provide a valuable resource for the ing from gene expression to cell cycle progression. DBC1 exploration of pathways involved in DBC1-associated has been linked to tumorigenesis both as an inhibitor of tumorigenesis. Molecular & Cellular Proteomics 15: histone deacetylases, HDAC3 and sirtuin 1, and as a tran- 10.1074/mcp.M115.054619, 791–809, 2016. scriptional cofactor for nuclear hormone receptors. How- ever, despite mounting interest in DBC1, relatively little is known about the range of its interacting partners and the Deleted in breast cancer 1 (DBC1)1 was first identified by scope of its functions. Here, we carried out a functional cloning a human chromosomal region observed to be ho- proteomics-based investigation of DBC1 interactions in mozygously deleted in multiple breast cancers (1). Having two relevant cell types, T cells and kidney cells. Micros- gained prominence as an important regulator of gene expres- copy, molecular biology, biochemistry, and mass spec- sion, DBC1 is now known to have additional functions in trometry studies allowed us to assess DBC1 mRNA and chromatin remodeling, transcriptional regulation, and modu- protein levels, localization, phosphorylation status, and protein interaction networks. -
Nuclear Perilipin 5 Integrates Lipid Droplet Lipolysis with PGC-1&Alpha
ARTICLE Received 28 Sep 2015 | Accepted 27 Jul 2016 | Published 24 Aug 2016 DOI: 10.1038/ncomms12723 OPEN Nuclear Perilipin 5 integrates lipid droplet lipolysis with PGC-1a/SIRT1-dependent transcriptional regulation of mitochondrial function Violeta I. Gallardo-Montejano1, Geetu Saxena2, Christine M. Kusminski3, Chaofeng Yang1, John L. McAfee1, Lisa Hahner1, Kathleen Hoch2, William Dubinsky2, Vihang A. Narkar2 & Perry E. Bickel1 Dysfunctional cellular lipid metabolism contributes to common chronic human diseases, including type 2 diabetes, obesity, fatty liver disease and diabetic cardiomyopathy. How cells balance lipid storage and mitochondrial oxidative capacity is poorly understood. Here we identify the lipid droplet protein Perilipin 5 as a catecholamine-triggered interaction partner of PGC-1a. We report that during catecholamine-stimulated lipolysis, Perilipin 5 is phosphory- lated by protein kinase A and forms transcriptional complexes with PGC-1a and SIRT1 in the nucleus. Perilipin 5 promotes PGC-1a co-activator function by disinhibiting SIRT1 deacetylase activity. We show by gain-and-loss of function studies in cells that nuclear Perilipin 5 promotes transcription of genes that mediate mitochondrial biogenesis and oxidative function. We propose that Perilipin 5 is an important molecular link that couples the coordinated catecholamine activation of the PKA pathway and of lipid droplet lipolysis with transcriptional regulation to promote efficient fatty acid catabolism and prevent mitochondrial dysfunction. 1 Division of Endocrinology, Department of Internal Medicine, The University of Texas Southwestern Medical Center, Dallas, Texas 75390, USA. 2 Center for Metabolic and Degenerative Diseases, The Brown Foundation Institute of Molecular Medicine for The Prevention Of Human Diseases, UT Health, Houston, Texas 77030, USA.