An Investigation of the CK2-Dependent Phosphoproteome Using Inhibitor Refractory CK2-Alpha
Total Page:16
File Type:pdf, Size:1020Kb
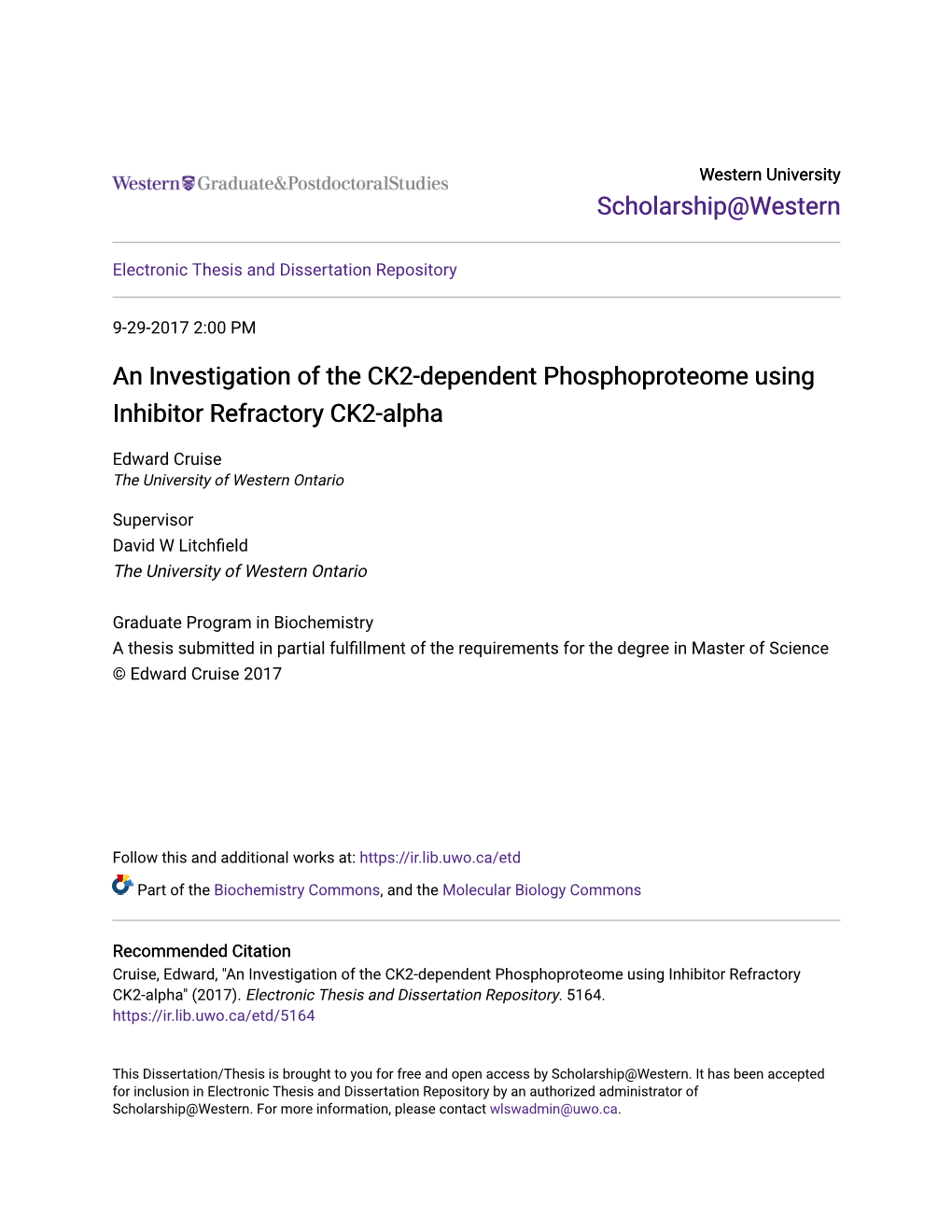
Load more
Recommended publications
-
Multiple Cellular Proteins Interact with LEDGF/P75 Through a Conserved Unstructured Consensus Motif
ARTICLE Received 19 Jan 2015 | Accepted 1 Jul 2015 | Published 6 Aug 2015 DOI: 10.1038/ncomms8968 Multiple cellular proteins interact with LEDGF/p75 through a conserved unstructured consensus motif Petr Tesina1,2,3,*, Katerˇina Cˇerma´kova´4,*, Magdalena Horˇejsˇ´ı3, Katerˇina Procha´zkova´1, Milan Fa´bry3, Subhalakshmi Sharma4, Frauke Christ4, Jonas Demeulemeester4, Zeger Debyser4, Jan De Rijck4,**, Va´clav Veverka1,** & Pavlı´na Rˇeza´cˇova´1,3,** Lens epithelium-derived growth factor (LEDGF/p75) is an epigenetic reader and attractive therapeutic target involved in HIV integration and the development of mixed lineage leukaemia (MLL1) fusion-driven leukaemia. Besides HIV integrase and the MLL1-menin complex, LEDGF/p75 interacts with various cellular proteins via its integrase binding domain (IBD). Here we present structural characterization of IBD interactions with transcriptional repressor JPO2 and domesticated transposase PogZ, and show that the PogZ interaction is nearly identical to the interaction of LEDGF/p75 with MLL1. The interaction with the IBD is maintained by an intrinsically disordered IBD-binding motif (IBM) common to all known cellular partners of LEDGF/p75. In addition, based on IBM conservation, we identify and validate IWS1 as a novel LEDGF/p75 interaction partner. Our results also reveal how HIV integrase efficiently displaces cellular binding partners from LEDGF/p75. Finally, the similar binding modes of LEDGF/p75 interaction partners represent a new challenge for the development of selective interaction inhibitors. 1 Institute of Organic Chemistry and Biochemistry of the ASCR, v.v.i., Flemingovo nam. 2, 166 10 Prague, Czech Republic. 2 Department of Genetics and Microbiology, Faculty of Science, Charles University in Prague, Vinicna 5, 128 44 Prague, Czech Republic. -
The Function and Evolution of C2H2 Zinc Finger Proteins and Transposons
The function and evolution of C2H2 zinc finger proteins and transposons by Laura Francesca Campitelli A thesis submitted in conformity with the requirements for the degree of Doctor of Philosophy Department of Molecular Genetics University of Toronto © Copyright by Laura Francesca Campitelli 2020 The function and evolution of C2H2 zinc finger proteins and transposons Laura Francesca Campitelli Doctor of Philosophy Department of Molecular Genetics University of Toronto 2020 Abstract Transcription factors (TFs) confer specificity to transcriptional regulation by binding specific DNA sequences and ultimately affecting the ability of RNA polymerase to transcribe a locus. The C2H2 zinc finger proteins (C2H2 ZFPs) are a TF class with the unique ability to diversify their DNA-binding specificities in a short evolutionary time. C2H2 ZFPs comprise the largest class of TFs in Mammalian genomes, including nearly half of all Human TFs (747/1,639). Positive selection on the DNA-binding specificities of C2H2 ZFPs is explained by an evolutionary arms race with endogenous retroelements (EREs; copy-and-paste transposable elements), where the C2H2 ZFPs containing a KRAB repressor domain (KZFPs; 344/747 Human C2H2 ZFPs) are thought to diversify to bind new EREs and repress deleterious transposition events. However, evidence of the gain and loss of KZFP binding sites on the ERE sequence is sparse due to poor resolution of ERE sequence evolution, despite the recent publication of binding preferences for 242/344 Human KZFPs. The goal of my doctoral work has been to characterize the Human C2H2 ZFPs, with specific interest in their evolutionary history, functional diversity, and coevolution with LINE EREs. -
A Collection of Pre-Mrna Splicing Mutants in Arabidopsis Thaliana
G3: Genes|Genomes|Genetics Early Online, published on April 7, 2020 as doi:10.1534/g3.119.400998 1 1 A collection of pre-mRNA splicing mutants in Arabidopsis thaliana 2 3 4 Tatsuo Kanno1,a, Peter Venhuizen2,a, Ming-Tsung Wu3, Phebe Chiou1, Chia-Liang Chang1, 5 Maria Kalyna2,c, Antonius J.M. Matzke1,c and Marjori Matzke1,c 6 7 1Institute of Plant and Microbial Biology, Academia Sinica, 128, Sec. 2, Academia Rd., Nangang 8 District, Taipei, 11529 Taiwan 9 10 2Department of Applied Genetics and Cell Biology, University of Natural Resources and Life 11 Sciences - BOKU, Muthgasse 18, 1190 Vienna, Austria 12 13 3Department of Plant Sciences, University of Cambridge, Downing Street, CB2 3EA Cambridge, 14 UK 15 16 athese authors should be regarded as joint first authors 17 18 19 cCorresponding authors: 20 Antonius J.M. Matzke ([email protected]) 21 Tel: +886-2787-1135 22 Marjori Matzke ([email protected]) 23 Tel: +886-2787-1135 24 Maria Kalyna ([email protected]) 25 Tel: +43-1-47654-94112 26 27 28 29 30 31 32 © The Author(s) 2020. Published by the Genetics Society of America. 2 33 34 Running title: Arabidopsis pre-mRNA splicing mutants 35 36 Key words: Arabidopsis thaliana, CBP80. miRNAs, mutant screen, pre-mRNA splicing 37 38 39 40 Corresponding authors: 41 Antonius J.M. Matzke ([email protected]), Institute of Plant and Microbial 42 Biology, Academia Sinica, 128, Sec. 2, Academia Rd., Nangang District, Taipei, 11529 Taiwan 43 Tel: +886-2787-1135 44 45 Marjori Matzke ([email protected]), Institute of Plant and Microbial Biology, 46 Academia Sinica, 128, Sec. -
IWS1 Antibody A
Revision 1 C 0 2 - t IWS1 Antibody a e r o t S Orders: 877-616-CELL (2355) [email protected] Support: 877-678-TECH (8324) 1 8 Web: [email protected] 6 www.cellsignal.com 5 # 3 Trask Lane Danvers Massachusetts 01923 USA For Research Use Only. Not For Use In Diagnostic Procedures. Applications: Reactivity: Sensitivity: MW (kDa): Source: UniProt ID: Entrez-Gene Id: WB, IP, IF-IC H M R Endogenous 140 Rabbit Q96ST2 55677 Product Usage Information Application Dilution Western Blotting 1:1000 Immunoprecipitation 1:50 Immunofluorescence (Immunocytochemistry) 1:200 Storage Supplied in 10 mM sodium HEPES (pH 7.5), 150 mM NaCl, 100 µg/ml BSA and 50% glycerol. Store at –20°C. Do not aliquot the antibody. Specificity / Sensitivity IWS1 Antibody detects endogenous levels of total IWS1 protein. Species Reactivity: Human, Mouse, Rat Source / Purification Polyclonal antibodies are produced by immunizing animals with a synthetic peptide corresponding to residues near the amino terminus of human IWS1 protein. Antibodies are purified by protein A and peptide affinity chromatography. Background Various steps in gene expression, such as mRNA processing, surveillance, export, and synthesis are coupled to transcription elongation (1,2). The C-terminal domain (CTD) of the large subunit of RNA polymerase II plays an important role in the integration of these different steps (1,2). IWS1 interacts with Spt6, a CTD-binding transcription elongation factor and H3 chaperone (1,2). IWS1 also recruits another CTD-binding protein, HYPB/Setd2 histone methyltransferase, to the RNA polymerase II complex for elongation-coupled H3K36 trimethylation (2). Thus, IWS1 links Spt6 and HYPB/Setd2 in a large complex and regulates mRNA synthesis and histone methylation at the co- transcriptional level (2). -
Dynamics of Transcription-Dependent H3k36me3 Marking by the SETD2:IWS1:SPT6 Ternary Complex
bioRxiv preprint doi: https://doi.org/10.1101/636084; this version posted May 14, 2019. The copyright holder for this preprint (which was not certified by peer review) is the author/funder. All rights reserved. No reuse allowed without permission. Dynamics of transcription-dependent H3K36me3 marking by the SETD2:IWS1:SPT6 ternary complex Katerina Cermakova1, Eric A. Smith1, Vaclav Veverka2, H. Courtney Hodges1,3,4,* 1 Department of Molecular & Cellular Biology, Center for Precision Environmental Health, and Dan L Duncan Comprehensive Cancer Center, Baylor College of Medicine, Houston, TX, 77030, USA 2 Institute of Organic Chemistry and Biochemistry, Czech Academy of Sciences, Prague, Czech Republic 3 Center for Cancer Epigenetics, The University of Texas MD Anderson Cancer Center, Houston, TX, 77030, USA 4 Department of Bioengineering, Rice University, Houston, TX, 77005, USA * Lead contact; Correspondence to: [email protected] Abstract The genome-wide distribution of H3K36me3 is maintained SETD2 contributes to gene expression by marking gene through various mechanisms. In human cells, H3K36 is bodies with H3K36me3, which is thought to assist in the mono- and di-methylated by eight distinct histone concentration of transcription machinery at the small portion methyltransferases; however, the predominant writer of the of the coding genome. Despite extensive genome-wide data trimethyl mark on H3K36 is SETD21,11,12. Interestingly, revealing the precise localization of H3K36me3 over gene SETD2 is a major tumor suppressor in clear cell renal cell bodies, the physical basis for the accumulation, carcinoma13, breast cancer14, bladder cancer15, and acute maintenance, and sharp borders of H3K36me3 over these lymphoblastic leukemias16–18. In these settings, mutations sites remains rudimentary. -
A Master Autoantigen-Ome Links Alternative Splicing, Female Predilection, and COVID-19 to Autoimmune Diseases
bioRxiv preprint doi: https://doi.org/10.1101/2021.07.30.454526; this version posted August 4, 2021. The copyright holder for this preprint (which was not certified by peer review) is the author/funder, who has granted bioRxiv a license to display the preprint in perpetuity. It is made available under aCC-BY 4.0 International license. A Master Autoantigen-ome Links Alternative Splicing, Female Predilection, and COVID-19 to Autoimmune Diseases Julia Y. Wang1*, Michael W. Roehrl1, Victor B. Roehrl1, and Michael H. Roehrl2* 1 Curandis, New York, USA 2 Department of Pathology, Memorial Sloan Kettering Cancer Center, New York, USA * Correspondence: [email protected] or [email protected] 1 bioRxiv preprint doi: https://doi.org/10.1101/2021.07.30.454526; this version posted August 4, 2021. The copyright holder for this preprint (which was not certified by peer review) is the author/funder, who has granted bioRxiv a license to display the preprint in perpetuity. It is made available under aCC-BY 4.0 International license. Abstract Chronic and debilitating autoimmune sequelae pose a grave concern for the post-COVID-19 pandemic era. Based on our discovery that the glycosaminoglycan dermatan sulfate (DS) displays peculiar affinity to apoptotic cells and autoantigens (autoAgs) and that DS-autoAg complexes cooperatively stimulate autoreactive B1 cell responses, we compiled a database of 751 candidate autoAgs from six human cell types. At least 657 of these have been found to be affected by SARS-CoV-2 infection based on currently available multi-omic COVID data, and at least 400 are confirmed targets of autoantibodies in a wide array of autoimmune diseases and cancer. -
Histone Methyltransferase Gene SETD2 Is a Novel Tumor Suppressor Gene in Clear Cell Renal Cell Carcinoma
Published OnlineFirst May 25, 2010; DOI: 10.1158/0008-5472.CAN-10-0120 Published OnlineFirst on May 25, 2010 as 10.1158/0008-5472.CAN-10-0120 Priority Report Cancer Research Histone Methyltransferase Gene SETD2 Is a Novel Tumor Suppressor Gene in Clear Cell Renal Cell Carcinoma Gerben Duns1, Eva van den Berg1, Inge van Duivenbode1, Jan Osinga1, Harry Hollema2, Robert M.W. Hofstra1, and Klaas Kok1 Abstract Sporadic clear cell renal cell carcinoma (cRCC) is genetically characterized by the recurrent loss of the short arm of chromosome 3, with a hotspot for copy number loss in the 3p21 region. We applied a method called “gene identification by nonsense-mediated mRNA decay inhibition” to a panel of 10 cRCC cell lines with 3p21 copy number loss to identify biallelic inactivated genes located at 3p21. This revealed inactivation of the histone methyltransferase gene SETD2, located on 3p21.31, as a common event in cRCC cells. SETD2 is nonredundantly responsible for trimethylation of the histone mark H3K36. Consistent with this function, we observed loss or a decrease of H3K36me3 in 7 out of the 10 cRCC cell lines. Identification of missense mutations in 2 out of 10 primary cRCC tumor samples added support to the involvement of loss of SETD2 function in the development of cRCC tumors. Cancer Res; 70(11); 4287–91. ©2010 AACR. Introduction the accumulation of PTC transcripts, which can be detected using gene expression microarrays. Several groups have suc- Clear cell renal cell carcinoma (cRCC) is the most preva- cessfully applied modified versions of the GINI method in their lent subtype of renal cell carcinoma and accounts for 3% of search for TSGs (7–9). -
1 1 Overexpression of the SETD2 WW Domain Inhibits the Phosphor-IWS1
bioRxiv preprint doi: https://doi.org/10.1101/2021.08.12.454141; this version posted August 12, 2021. The copyright holder for this preprint (which was not certified by peer review) is the author/funder, who has granted bioRxiv a license to display the preprint in perpetuity. It is made available under aCC-BY-NC 4.0 International license. Laliotis et al., SETD2 WW domain inhibits the IWS1 RNA splicing program 1 2 Overexpression of the SETD2 WW domain inhibits the phosphor-IWS1/SETD2 3 interaction and the oncogenic AKT/IWS1 RNA splicing program. 4 5 6 Georgios I. Laliotis1,2,3,8*, Evangelia Chavdoula1,2, Vollter Anastas1,2,4, Satishkumar Singh5, 7 Adam D. Kenney6,7, Samir Achaya1,2,9, Jacob S. Yount6,7, Lalit Sehgal2,5 and Philip N. 8 Tsichlis1,2,* 9 10 1The Ohio State University, Department of Cancer Biology and Genetics, Columbus, OH, 43210, 2The Ohio State 11 University Comprehensive Cancer Center-Arthur G. James Cancer Hospital and Richard J. Solove Research 12 Institute, Columbus, OH, 43210, 3University of Crete, School of Medicine, Heraklion Crete, 71500, Greece, 4Tufts 13 Graduate School of Biomedical Sciences, Program in Genetics, Boston, MA, 02111, USA, 5College of Medicine, 14 Department of Hematology, The Ohio State University, Columbus, OH 43210, 7Department of Microbial Infection 15 and Immunity, The Ohio State University, Columbus, OH, 43210 16 17 Running Title: SETD2 WW domain inhibits the IWS1 RNA splicing program 18 19 20 Present address : 21 8Department of Oncology, Sidney Kimmel Comprehensive Cancer Center, Johns Hopkins School of Medicine, 22 Baltimore, MD, 21231 23 9Nationswide Children's Hospital, Columbus, OH, 43205 24 25 *Correspondence should be addressed to: Philip N. -
AKT3-Mediated IWS1 Phosphorylation Promotes the Proliferation of EGFR-Mutant Lung Adenocarcinomas Through Cell Cycle-Regulated U2AF2 RNA Splicing ✉ Georgios I
ARTICLE https://doi.org/10.1038/s41467-021-24795-1 OPEN AKT3-mediated IWS1 phosphorylation promotes the proliferation of EGFR-mutant lung adenocarcinomas through cell cycle-regulated U2AF2 RNA splicing ✉ Georgios I. Laliotis 1,2,3,11 , Evangelia Chavdoula1,2, Maria D. Paraskevopoulou4, Abdul Kaba1,2, Alessandro La Ferlita1,2,5, Satishkumar Singh2,6, Vollter Anastas 1,2,7, Keith A. Nair II 1,2, Arturo Orlacchio1,2, Vasiliki Taraslia4,12, Ioannis Vlachos8,13, Marina Capece1,2, Artemis Hatzigeorgiou8, Dario Palmieri1,2, 1234567890():,; Christos Tsatsanis3,9, Salvatore Alaimo 5, Lalit Sehgal 2,6, David P. Carbone 2,10, Vincenzo Coppola 1,2 & ✉ Philip N. Tsichlis 1,2,7 AKT-phosphorylated IWS1 regulates alternative RNA splicing via a pathway that is active in lung cancer. RNA-seq studies in lung adenocarcinoma cells lacking phosphorylated IWS1, identified a exon 2-deficient U2AF2 splice variant. Here, we show that exon 2 inclusion in the U2AF2 mRNA is a cell cycle-dependent process that is regulated by LEDGF/SRSF1 splicing complexes, whose assembly is controlled by the IWS1 phosphorylation-dependent deposition of histone H3K36me3 marks in the body of target genes. The exon 2-deficient U2AF2 mRNA encodes a Serine-Arginine-Rich (RS) domain-deficient U2AF65, which is defective in CDCA5 pre-mRNA processing. This results in downregulation of the CDCA5-encoded protein Sororin, a phosphorylation target and regulator of ERK, G2/M arrest and impaired cell proliferation and tumor growth. Analysis of human lung adenocarcinomas, confirmed activation of the pathway in EGFR-mutant tumors and showed that pathway activity correlates with tumor stage, histologic grade, metastasis, relapse after treatment, and poor prognosis. -
Alm Thesis Post Defense
Characterization of the Association of mRNA Export Factor Yra1 with the C-terminal Domain of RNA Polymerase II in vivo and in vitro by April Lee MacKellar Department of Biochemistry Duke University Date:_______________________ Approved: ___________________________ Arno Greenleaf, Supervisor ___________________________ Kenneth Kreuzer ___________________________ Michael Been ___________________________ Laura Rusche ___________________________ Mariano Garcia-Blanco Dissertation suBmitted in partial fulfillment of the requirements for the degree of Doctor of Philosophy in the Department of Biochemistry in the Graduate School of Duke University 2011 i v ABSTRACT Characterization of the Association of mRNA Export Factor Yra1 with the C-terminal Domain of RNA Polymerase II in vivo and in vitro by April Lee MacKellar Department of Biochemistry Duke University Date:_______________________ Approved: ___________________________ Arno Greenleaf, Supervisor ___________________________ Kenneth Kreuzer ___________________________ Michael Been ___________________________ Laura Rusche ___________________________ Mariano Garcia-Blanco An aBstract of a dissertation suBmitted in partial fulfillment of the requirements for the degree of Doctor of Philosophy in the Department of Biochemistry in the Graduate School of Duke University 2011 i v Copyright By April Lee MacKellar 2011 Abstract The unique C-terminal domain (CTD) of RNA polymerase II (RNAPII), composed of tandem heptad repeats of the consensus sequence YSPTSPS, is subject to differential phosphorylation throughout the transcription cycle. Several RNA processing factors have been shown to bind the appropriately phosphorylated CTD, and this facilitates their localization to nascent pre-mRNA during transcription. In Saccharomyces cerevisiae, the mRNA export protein Yra1 (ALY/REF in metazoa) has been shown to cotranscriptionally associate with mRNA and is thought to deliver it to the nuclear pore complex for export to the cytoplasm. -
Supplementary Material
Supplementary material Supplementary figures Supplementary Figure 1. 2,566-features miRNA-transcriptomic dataset signature performance, selected predictors and model inspection: A. Receiver Operating Characteristic (ROC) Curve for AD class, B. Precision-recall plot, C. Probabilities density plot, D. Supervised Principal Component Analysis plot depicting discrimination between AD patients and age-sex matched cognitively healthy individuals, E. Cumulative feature importance plot of the 25 miRNA predictors of the signature. 1 Supplementary Figure 2. 2,566-features miRNA-transcriptomic sub-dataset (train) signature performance, selected predictors and model inspection: A. Receiver Operating Characteristic (ROC) Curve for AD class, B. Precision-recall plot, C. Probabilities density plot, D. Supervised Principal Component Analysis plot depicting discrimination between AD patients and age-sex matched cognitively healthy individuals, E. Cumulative feature importance plot of the 25 miRNA predictors of the signature. Supplementary Figure 3. Validation of 2,566-features miRNA sub-dataset (test) signature performance: A. Receiver Operating Characteristic (ROC) Curve for AD class, B. Precision-recall plot, C. Supervised Principal Component Analysis plot depicting discrimination between AD patients and age-sex matched cognitively healthy individuals in test dataset. Supplementary Figure 4. 32,053-features mRNA-transcriptomic dataset signature performance, selected predictors and model inspection: A. Receiver Operating Characteristic (ROC) Curve for AD class, B. Precision-recall plot, C. Probabilities density plot, D. Supervised Principal Component Analysis plot depicting discrimination between AD patients and age-sex matched cognitively healthy individuals, E. Cumulative feature importance plot of the 25 mRNA predictors of the reference signature. Supplementary Figure 5. 32,053-features mRNA-transcriptomic sub-dataset (train) signature performance, selected predictors and model inspection: A. -
Arabidopsis IWS1 Interacts with Transcription Factor BES1 and Is Involved in Plant Steroid Hormone Brassinosteroid Regulated Gene Expression
Arabidopsis IWS1 interacts with transcription factor BES1 and is involved in plant steroid hormone brassinosteroid regulated gene expression Lei Li, Huaxun Ye, Hongqing Guo, and Yanhai Yin1 Department of Genetics, Development and Cell Biology, Iowa State University, Ames, IA 50011 Edited by Mark Estelle, The University of California San Diego, La Jolla, CA, and approved January 08, 2010 (received for review August 13, 2009) Plant steroid hormones, brassinosteroids (BRs), regulate essential knowledge about the transcriptional mechanisms by which BES1 growth and developmental processes. BRs signal throughmembrane- and BZR1 regulate gene expression is still limited. localized receptor BRI1 and several other signaling components to IWS1/SPN1 protein was firstly identified in yeast (13, 14). It was regulate the BES1 and BZR1 family transcription factors, which in turn reported to be involved in postrecruitment of RNAPII-mediated control the expression of hundreds of target genes. However, knowl- transcription and required for the expression of several inducible edge about the transcriptional mechanisms by which BES1/BZR1 genes (14). It was also copurified with RNAPII and several tran- regulate gene expression is limited. By a forward genetic approach, scription elongation factors, including Spt6, Spt4/Spt5, and TFIIS we have discovered that Arabidopsis thaliana Interact-With-Spt6 (13, 15, 16). Recent studies indicated that the yeast IWS1/SPN1 (AtIWS1), an evolutionarily conservedproteinimplicatedinRNApoly- recruited Spt6 and the SWI/SNF chromatin remodeling complex merase II (RNAPII) postrecruitment and transcriptional elongation pro- during transcriptional activation (17). Human IWS1 has been cesses, is required for BR-induced gene expression. Loss-of-function shown to facilitate mRNA export and to coordinate Spt6 and the mutations in AtIWS1 lead to overall dwarfism in Arabidopsis, reduced histone methyltransferase HYBP/Setd2 to regulate histone mod- BR response, genome-wide decrease in BR-induced gene expression, ifications (18, 19).