Enzyme Activities in Soils As Affected by Management Practices Mine Ekenler Iowa State University
Total Page:16
File Type:pdf, Size:1020Kb
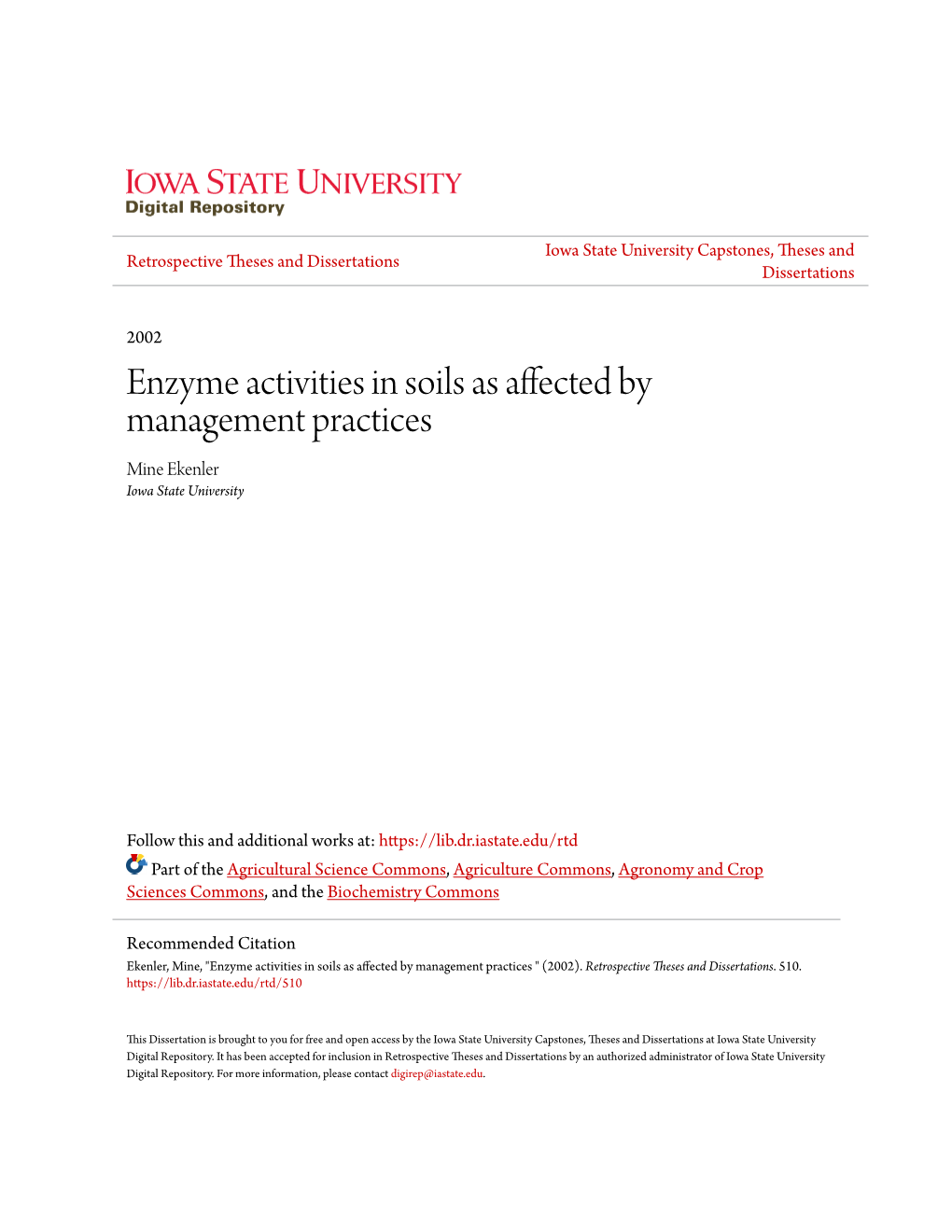
Load more
Recommended publications
-
Identification and Characterization of a Mammalian 14-Kda
Eur. J. Biochem. 269, 5016–5023 (2002) Ó FEBS 2002 doi:10.1046/j.1432-1033.2002.03206.x Identification and characterization of a mammalian 14-kDa phosphohistidine phosphatase Pia Ek1, Gunilla Pettersson1,BoEk2, Feng Gong1, Jin-Ping Li1 and O¨ rjan Zetterqvist1 1Department of Medical Biochemistry and Microbiology, Uppsala University, Uppsala, Sweden; 2Department of Plant Biology, The Swedish University of Agricultural Sciences, Uppsala, Sweden Protein histidine phosphorylation in eukaryotes has been cloning from a human embryonic kidney cell cDNA- sparsely studied compared to protein serine/threonine and library followed by expression and purification, yielded a tyrosine phosphorylation. In an attempt to rectify this by protein with a molecular mass of 13 700 Da, and an probing porcine liver cytosol with the phosphohistidine- EDTA-insensitive phosphohistidine phosphatase activity containing peptide succinyl-Ala-His(P)-Pro-Phe-p-nitro- of 9 lmolÆmin)1Æmg)1 towards phosphopeptide I. No anilide (phosphopeptide I), we observed a phosphatase detectable activity was obtained towards a set of phos- activity that was insensitive towards okadaic acid and phoserine-, phosphothreonine-, and phosphotyrosine pep- EDTA. This suggested the existence of a phosphohistidine tides. Northern blot analysis indicated that the human phosphatase different from protein phosphatase 1, 2A phosphohistidine phosphatase mRNA was present pre- and 2C. A 1000-fold purification to apparent homogeneity ferentially in heart and skeletal muscle. These results gave a 14-kDa phosphatase with a specific activity of 3 provide a new tool for studying eukaryotic histidine )1 )1 lmolÆmin Æmg at pH 7.5 with 7 lM phosphopeptide I phosphorylation/dephosphorylation. as substrate. Partial amino-acid sequence determination of Keywords: dephosphorylation; N-phosphorylation; phos- the purified porcine enzyme by MS revealed similarity phoamidase; phosphopeptide; protein histidine phospha- with a human sequence representing a human chromo- tase. -
Natural Products Containing 'Rare'
molecules Review Natural Products Containing ‘Rare’ Organophosphorus Functional Groups Janusz J. Petkowski 1,* , William Bains 2 and Sara Seager 1,3,4 1 Department of Earth, Atmospheric, and Planetary Sciences, Massachusetts Institute of Technology, 77 Mass. Ave., Cambridge, MA 02139, USA; [email protected] 2 Rufus Scientific, 37 The Moor, Melbourn, Royston, Herts SG8 6ED, UK; [email protected] 3 Department of Physics, Massachusetts Institute of Technology, 77 Mass. Ave., Cambridge, MA 02139, USA 4 Department of Aeronautics and Astronautics, Massachusetts Institute of Technology, 77 Mass. Ave., Cambridge, MA 02139, USA * Correspondence: [email protected] Received: 21 January 2019; Accepted: 22 February 2019; Published: 28 February 2019 Abstract: Phosphorous-containing molecules are essential constituents of all living cells. While the phosphate functional group is very common in small molecule natural products, nucleic acids, and as chemical modification in protein and peptides, phosphorous can form P–N (phosphoramidate), P–S (phosphorothioate), and P–C (e.g., phosphonate and phosphinate) linkages. While rare, these moieties play critical roles in many processes and in all forms of life. In this review we thoroughly categorize P–N, P–S, and P–C natural organophosphorus compounds. Information on biological source, biological activity, and biosynthesis is included, if known. This review also summarizes the role of phosphorylation on unusual amino acids in proteins (N- and S-phosphorylation) and reviews the natural phosphorothioate (P–S) and phosphoramidate (P–N) modifications of DNA and nucleotides with an emphasis on their role in the metabolism of the cell. We challenge the commonly held notion that nonphosphate organophosphorus functional groups are an oddity of biochemistry, with no central role in the metabolism of the cell. -
Natural Products Containing 'Rare'
Natural Products Containing ‘Rare’ Organophosphorus Functional Groups The MIT Faculty has made this article openly available. Please share how this access benefits you. Your story matters. Citation Petkowski, Janusz, et al. “Natural Products Containing ‘Rare’ Organophosphorus Functional Groups.” Molecules, vol. 24, no. 5, Feb. 2019, p. 866. As Published http://dx.doi.org/10.3390/molecules24050866 Publisher Multidisciplinary Digital Publishing Institute Version Final published version Citable link http://hdl.handle.net/1721.1/120918 Terms of Use Creative Commons Attribution Detailed Terms https://creativecommons.org/licenses/by/4.0/ molecules Review Natural Products Containing ‘Rare’ Organophosphorus Functional Groups Janusz J. Petkowski 1,* , William Bains 2 and Sara Seager 1,3,4 1 Department of Earth, Atmospheric, and Planetary Sciences, Massachusetts Institute of Technology, 77 Mass. Ave., Cambridge, MA 02139, USA; [email protected] 2 Rufus Scientific, 37 The Moor, Melbourn, Royston, Herts SG8 6ED, UK; [email protected] 3 Department of Physics, Massachusetts Institute of Technology, 77 Mass. Ave., Cambridge, MA 02139, USA 4 Department of Aeronautics and Astronautics, Massachusetts Institute of Technology, 77 Mass. Ave., Cambridge, MA 02139, USA * Correspondence: [email protected] Received: 21 January 2019; Accepted: 22 February 2019; Published: 28 February 2019 Abstract: Phosphorous-containing molecules are essential constituents of all living cells. While the phosphate functional group is very common in small molecule natural products, nucleic acids, and as chemical modification in protein and peptides, phosphorous can form P–N (phosphoramidate), P–S (phosphorothioate), and P–C (e.g., phosphonate and phosphinate) linkages. While rare, these moieties play critical roles in many processes and in all forms of life. -
Advances in Development of New Tools for the Study of Phosphohistidine Mehul V Makwana1,2, Richmond Muimo2 and Richard FW Jackson1
Laboratory Investigation (2017), 1–13 © 2017 USCAP, Inc All rights reserved 0023-6837/17 $32.00 PATHOBIOLOGY IN FOCUS Corrected Advances in development of new tools for the study of phosphohistidine Mehul V Makwana1,2, Richmond Muimo2 and Richard FW Jackson1 Protein phosphorylation is an important post-translational modification that is an integral part of cellular function. The O-phosphorylated amino-acid residues, such as phosphoserine (pSer), phosphothreonine (pThr) and phosphotyrosine (pTyr), have dominated the literature while the acid labile N-linked phosphorylated amino acids, such as phosphohistidine (pHis), have largely been historically overlooked because of the acidic conditions routinely used in amino-acid detection and analysis. This review highlights some misinterpretations that have arisen in the existing literature, pinpoints outstanding questions and potential future directions to clarify the role of pHis in mammalian signalling systems. Particular emphasis is placed on pHis isomerization and the hybrid functionality for both pHis and pTyr of the proposed τ-pHis analogue bearing the triazole residue. Laboratory Investigation advance online publication, 4 December 2017; doi:10.1038/labinvest.2017.126 Protein phosphorylation is one of the most commonly chemical properties: first, unlike other phosphorylated studied post-translational modifications. In general, the residues there are two isomers of pHis; τ- and π-pHis, both phosphorylation of any amino-acid residue results in a of which are found in nature (Figure 1).3,4 The τ- and π-pHis change in charge and thus in the protein surface potential. are chemically different from each other in both structure, For example, as phosphoryl groups exist mostly as a dianion reactivity and stability under certain conditions (vide infra). -
O O2 Enzymes Available from Sigma Enzymes Available from Sigma
COO 2.7.1.15 Ribokinase OXIDOREDUCTASES CONH2 COO 2.7.1.16 Ribulokinase 1.1.1.1 Alcohol dehydrogenase BLOOD GROUP + O O + O O 1.1.1.3 Homoserine dehydrogenase HYALURONIC ACID DERMATAN ALGINATES O-ANTIGENS STARCH GLYCOGEN CH COO N COO 2.7.1.17 Xylulokinase P GLYCOPROTEINS SUBSTANCES 2 OH N + COO 1.1.1.8 Glycerol-3-phosphate dehydrogenase Ribose -O - P - O - P - O- Adenosine(P) Ribose - O - P - O - P - O -Adenosine NICOTINATE 2.7.1.19 Phosphoribulokinase GANGLIOSIDES PEPTIDO- CH OH CH OH N 1 + COO 1.1.1.9 D-Xylulose reductase 2 2 NH .2.1 2.7.1.24 Dephospho-CoA kinase O CHITIN CHONDROITIN PECTIN INULIN CELLULOSE O O NH O O O O Ribose- P 2.4 N N RP 1.1.1.10 l-Xylulose reductase MUCINS GLYCAN 6.3.5.1 2.7.7.18 2.7.1.25 Adenylylsulfate kinase CH2OH HO Indoleacetate Indoxyl + 1.1.1.14 l-Iditol dehydrogenase L O O O Desamino-NAD Nicotinate- Quinolinate- A 2.7.1.28 Triokinase O O 1.1.1.132 HO (Auxin) NAD(P) 6.3.1.5 2.4.2.19 1.1.1.19 Glucuronate reductase CHOH - 2.4.1.68 CH3 OH OH OH nucleotide 2.7.1.30 Glycerol kinase Y - COO nucleotide 2.7.1.31 Glycerate kinase 1.1.1.21 Aldehyde reductase AcNH CHOH COO 6.3.2.7-10 2.4.1.69 O 1.2.3.7 2.4.2.19 R OPPT OH OH + 1.1.1.22 UDPglucose dehydrogenase 2.4.99.7 HO O OPPU HO 2.7.1.32 Choline kinase S CH2OH 6.3.2.13 OH OPPU CH HO CH2CH(NH3)COO HO CH CH NH HO CH2CH2NHCOCH3 CH O CH CH NHCOCH COO 1.1.1.23 Histidinol dehydrogenase OPC 2.4.1.17 3 2.4.1.29 CH CHO 2 2 2 3 2 2 3 O 2.7.1.33 Pantothenate kinase CH3CH NHAC OH OH OH LACTOSE 2 COO 1.1.1.25 Shikimate dehydrogenase A HO HO OPPG CH OH 2.7.1.34 Pantetheine kinase UDP- TDP-Rhamnose 2 NH NH NH NH N M 2.7.1.36 Mevalonate kinase 1.1.1.27 Lactate dehydrogenase HO COO- GDP- 2.4.1.21 O NH NH 4.1.1.28 2.3.1.5 2.1.1.4 1.1.1.29 Glycerate dehydrogenase C UDP-N-Ac-Muramate Iduronate OH 2.4.1.1 2.4.1.11 HO 5-Hydroxy- 5-Hydroxytryptamine N-Acetyl-serotonin N-Acetyl-5-O-methyl-serotonin Quinolinate 2.7.1.39 Homoserine kinase Mannuronate CH3 etc. -
(12) Patent Application Publication (10) Pub. No.: US 2012/0266329 A1 Mathur Et Al
US 2012026.6329A1 (19) United States (12) Patent Application Publication (10) Pub. No.: US 2012/0266329 A1 Mathur et al. (43) Pub. Date: Oct. 18, 2012 (54) NUCLEICACIDS AND PROTEINS AND CI2N 9/10 (2006.01) METHODS FOR MAKING AND USING THEMI CI2N 9/24 (2006.01) CI2N 9/02 (2006.01) (75) Inventors: Eric J. Mathur, Carlsbad, CA CI2N 9/06 (2006.01) (US); Cathy Chang, San Marcos, CI2P 2L/02 (2006.01) CA (US) CI2O I/04 (2006.01) CI2N 9/96 (2006.01) (73) Assignee: BP Corporation North America CI2N 5/82 (2006.01) Inc., Houston, TX (US) CI2N 15/53 (2006.01) CI2N IS/54 (2006.01) CI2N 15/57 2006.O1 (22) Filed: Feb. 20, 2012 CI2N IS/60 308: Related U.S. Application Data EN f :08: (62) Division of application No. 1 1/817,403, filed on May AOIH 5/00 (2006.01) 7, 2008, now Pat. No. 8,119,385, filed as application AOIH 5/10 (2006.01) No. PCT/US2006/007642 on Mar. 3, 2006. C07K I4/00 (2006.01) CI2N IS/II (2006.01) (60) Provisional application No. 60/658,984, filed on Mar. AOIH I/06 (2006.01) 4, 2005. CI2N 15/63 (2006.01) Publication Classification (52) U.S. Cl. ................... 800/293; 435/320.1; 435/252.3: 435/325; 435/254.11: 435/254.2:435/348; (51) Int. Cl. 435/419; 435/195; 435/196; 435/198: 435/233; CI2N 15/52 (2006.01) 435/201:435/232; 435/208; 435/227; 435/193; CI2N 15/85 (2006.01) 435/200; 435/189: 435/191: 435/69.1; 435/34; CI2N 5/86 (2006.01) 435/188:536/23.2; 435/468; 800/298; 800/320; CI2N 15/867 (2006.01) 800/317.2: 800/317.4: 800/320.3: 800/306; CI2N 5/864 (2006.01) 800/312 800/320.2: 800/317.3; 800/322; CI2N 5/8 (2006.01) 800/320.1; 530/350, 536/23.1: 800/278; 800/294 CI2N I/2 (2006.01) CI2N 5/10 (2006.01) (57) ABSTRACT CI2N L/15 (2006.01) CI2N I/19 (2006.01) The invention provides polypeptides, including enzymes, CI2N 9/14 (2006.01) structural proteins and binding proteins, polynucleotides CI2N 9/16 (2006.01) encoding these polypeptides, and methods of making and CI2N 9/20 (2006.01) using these polynucleotides and polypeptides. -
(12) Patent Application Publication (10) Pub. No.: US 2015/0240226A1 Mathur Et Al
US 20150240226A1 (19) United States (12) Patent Application Publication (10) Pub. No.: US 2015/0240226A1 Mathur et al. (43) Pub. Date: Aug. 27, 2015 (54) NUCLEICACIDS AND PROTEINS AND CI2N 9/16 (2006.01) METHODS FOR MAKING AND USING THEMI CI2N 9/02 (2006.01) CI2N 9/78 (2006.01) (71) Applicant: BP Corporation North America Inc., CI2N 9/12 (2006.01) Naperville, IL (US) CI2N 9/24 (2006.01) CI2O 1/02 (2006.01) (72) Inventors: Eric J. Mathur, San Diego, CA (US); CI2N 9/42 (2006.01) Cathy Chang, San Marcos, CA (US) (52) U.S. Cl. CPC. CI2N 9/88 (2013.01); C12O 1/02 (2013.01); (21) Appl. No.: 14/630,006 CI2O I/04 (2013.01): CI2N 9/80 (2013.01); CI2N 9/241.1 (2013.01); C12N 9/0065 (22) Filed: Feb. 24, 2015 (2013.01); C12N 9/2437 (2013.01); C12N 9/14 Related U.S. Application Data (2013.01); C12N 9/16 (2013.01); C12N 9/0061 (2013.01); C12N 9/78 (2013.01); C12N 9/0071 (62) Division of application No. 13/400,365, filed on Feb. (2013.01); C12N 9/1241 (2013.01): CI2N 20, 2012, now Pat. No. 8,962,800, which is a division 9/2482 (2013.01); C07K 2/00 (2013.01); C12Y of application No. 1 1/817,403, filed on May 7, 2008, 305/01004 (2013.01); C12Y 1 1 1/01016 now Pat. No. 8,119,385, filed as application No. PCT/ (2013.01); C12Y302/01004 (2013.01); C12Y US2006/007642 on Mar. 3, 2006. -
Springer Handbook of Enzymes
Dietmar Schomburg Ida Schomburg (Eds.) Springer Handbook of Enzymes Alphabetical Name Index 1 23 © Springer-Verlag Berlin Heidelberg New York 2010 This work is subject to copyright. All rights reserved, whether in whole or part of the material con- cerned, specifically the right of translation, printing and reprinting, reproduction and storage in data- bases. The publisher cannot assume any legal responsibility for given data. Commercial distribution is only permitted with the publishers written consent. Springer Handbook of Enzymes, Vols. 1–39 + Supplements 1–7, Name Index 2.4.1.60 abequosyltransferase, Vol. 31, p. 468 2.7.1.157 N-acetylgalactosamine kinase, Vol. S2, p. 268 4.2.3.18 abietadiene synthase, Vol. S7,p.276 3.1.6.12 N-acetylgalactosamine-4-sulfatase, Vol. 11, p. 300 1.14.13.93 (+)-abscisic acid 8’-hydroxylase, Vol. S1, p. 602 3.1.6.4 N-acetylgalactosamine-6-sulfatase, Vol. 11, p. 267 1.2.3.14 abscisic-aldehyde oxidase, Vol. S1, p. 176 3.2.1.49 a-N-acetylgalactosaminidase, Vol. 13,p.10 1.2.1.10 acetaldehyde dehydrogenase (acetylating), Vol. 20, 3.2.1.53 b-N-acetylgalactosaminidase, Vol. 13,p.91 p. 115 2.4.99.3 a-N-acetylgalactosaminide a-2,6-sialyltransferase, 3.5.1.63 4-acetamidobutyrate deacetylase, Vol. 14,p.528 Vol. 33,p.335 3.5.1.51 4-acetamidobutyryl-CoA deacetylase, Vol. 14, 2.4.1.147 acetylgalactosaminyl-O-glycosyl-glycoprotein b- p. 482 1,3-N-acetylglucosaminyltransferase, Vol. 32, 3.5.1.29 2-(acetamidomethylene)succinate hydrolase, p. 287 Vol. -
Arylamidase Activity of Soils Verónica Acosta-Martínez Iowa State University
Iowa State University Capstones, Theses and Retrospective Theses and Dissertations Dissertations 2000 Arylamidase activity of soils Verónica Acosta-Martínez Iowa State University Follow this and additional works at: https://lib.dr.iastate.edu/rtd Part of the Agriculture Commons, and the Soil Science Commons Recommended Citation Acosta-Martínez, Verónica, "Arylamidase activity of soils " (2000). Retrospective Theses and Dissertations. 12500. https://lib.dr.iastate.edu/rtd/12500 This Dissertation is brought to you for free and open access by the Iowa State University Capstones, Theses and Dissertations at Iowa State University Digital Repository. It has been accepted for inclusion in Retrospective Theses and Dissertations by an authorized administrator of Iowa State University Digital Repository. For more information, please contact [email protected]. INFORMATION TO USERS This manuscript has been reproduced from the microfilm master. UMI films the text directly from the original or copy submitted. Thus, some thesis and dissertation copies are in typewriter face, while others may be from any type of computer printer. The quality of this reproduction is dependent upon the quality of the copy submitted. Broken or indistinct print, colored or poor quality illustrations and photographs, print bleedthrough, substandard margins, and improper alignment can adversely affect reproduction. In the unlikely event that the author dkJ not send UMI a complete manuscript and there are missing pages, these will be noted. Also, if unauthorized copyright material had to be removed, a note will indicate the deletion. Oversize materials (e.g., maps, drawings, charts) are reproduced by sectioning the original, beginning at the upper left-hand comer and continuing from left to right in equal sections with small overiaps. -
Comparative Genomics and Metabolomics Analysis Of
www.nature.com/scientificreports OPEN Comparative genomics and metabolomics analysis of Riemerella anatipestifer strain CH‑1 and CH‑2 Jibin Liu1,2,3,4, Anchun Cheng1,2,3,4*, Mingshu Wang1,2,3*, Mafeng Liu1,2,3, Dekang Zhu2,3, Qiao Yang1,2,3, Ying Wu1,2,3, Renyong Jia1,2,3, Shun Chen1,2,3, Xinxin Zhao1,2,3, Shaqiu Zhang1,2,3, Juan Huang1,2,3, Xumin Ou1,2,3, Sai Mao1,2,3, Qun Gao1,2,3, Xingjian Wen1,2,3, Ling Zhang1,2,3, Yunya Liu1,2,3, Yanling Yu1,2,3, Bin Tian1,3, Leichang Pan1,3, Mujeeb Ur Rehman1,3 & Xiaoyue Chen2,3 Riemerella anatipestifer is a major pathogenic microorganism in poultry causing serositis with signifcant mortality. Serotype 1 and 2 were most pathogenic, prevalent, and liable over the world. In this study, the intracellular metabolites in R. anatipestifer strains RA‑CH‑1 (serotype 1) and RA‑CH‑2 (serotype 2) were identifed by gas chromatography‑mass spectrometer (GC–MS). The metabolic profles were performed using hierarchical clustering and partial least squares discriminant analysis (PLS‑DA). The results of hierarchical cluster analysis showed that the amounts of the detected metabolites were more abundant in RA‑CH‑2. RA‑CH‑1 and RA‑CH‑2 were separated by the PLS‑DA model. 24 potential biomarkers participated in nine metabolisms were contributed predominantly to the separation. Based on the complete genome sequence database and metabolite data, the frst large‑scale metabolic models of iJL463 (RA‑CH‑1) and iDZ470 (RA‑CH‑2) were reconstructed. In addition, we explained the change of purine metabolism combined with the transcriptome and metabolomics data. -
Bovine Liver Phosphoamidase As a Protein Histidine/Lysine Phosphatase
J. Biochem. 126, 368-374 (1999) Bovine Liver Phosphoamidase as a Protein Histidine/Lysine Phosphatase Hiroyuki Hiraishi, Fumiaki Yokoi, and Akira Kumon1 Department of Biochemistry, Saga Medical School, Nabeshima 5-1-1, Saga, Saga 849-8501 Received March 8, 1999; accepted June 1, 1999 A 13-kDa phosphoamidase was isolated as a single band on SDS-PAGE from bovine liver. Its Stokes' radius, sedimentation coefficient, molecular mass, and optimal pH were estimated to be 1.6nm, 1.8s, 13kDa, and 6.5, respectively. The enzyme released P, from 3-phosphohistidine, 6-phospholysine, and amidophosphate at rates of 0.9, 0.6, and 2.6 ƒÊ mol/min/mg protein, respectively. However, it did not dephosphorylate phosphocrea tine, NƒÖ-phosphoarginine, imidodiphosphate, or O-phosphorylated compounds including inorganic pyrophosphate. It also dephosphorylated succinic thiokinase and nucleoside diphosphate kinase autophosphorylated at His residues, indicating that it works as a protein histidine phosphatase. A thiol reagent, 30ƒÊM N-ethylmaleimide, depressed the activity by half, while a thiol compound, 2-mercaptoethanol, protected the enzyme from heat-inactivation. Five millimolar divalent cations, such as Mg2+ and Mn2+, and 5mM EDTA, had no effect on the activity. Key words: characterization, nucleoside diphosphate kinase, protein histidine/lysine phosphatase, purification, succinic thiokinase. Phosphoamidases are enzymes that hydrolyze the nitrogen- phoamidase that is specific only for P-Arg and is free of the phosphorus bond (N-P bond) of given N-phosphorylated activity that hydrolyzes O-phosphorylated compounds and compounds. The phosphoamidase classified as [EC 3.9.1.1] N-phosphorylated compounds including P-His, P-Lys, NP, dephosphorylates phosphocreatine and NƒÖ-phosphoargi and phosphocreatine (7, 8). -
Phosphorus Compounds of Natural Origin: Prebiotic, Stereochemistry, Application
S S symmetry Review Phosphorus Compounds of Natural Origin: Prebiotic, Stereochemistry, Application Oleg I. Kolodiazhnyi V.P. Kukhar’ Institute of Bioorganic Chemistry and Petrochemistry, NAS of Ukraine, Murmanska st., 1, 02094 Kyiv, Ukraine; [email protected] Abstract: Organophosphorus compounds play a vital role as nucleic acids, nucleotide coenzymes, metabolic intermediates and are involved in many biochemical processes. They are part of DNA, RNA, ATP and a number of important biological elements of living organisms. Synthetic compounds of this class have found practical application as agrochemicals, pharmaceuticals, bioregulators, and othrs. In recent years, a large number of phosphorus compounds containing P-O, P-N, P-C bonds have been isolated from natural sources. Many of them have shown interesting biological properties and have become the objects of intensive scientific research. Most of these compounds contain asymmetric centers, the absolute configurations of which have a significant effect on the biological properties of the products of their transformations. This area of research on natural phosphorus compounds is still little-studied, that prompted us to analyze and discuss it in our review. Moreover natural organophosphorus compounds represent interesting models for the development of new biologically active compounds, and a number of promising drugs and agrochemicals have already been obtained on their basis. The review also discusses the history of the development of ideas about the role of organophosphorus compounds and stereochemistry in the origin of life on Earth, starting from the prebiotic period, that allows us in a new way to consider this most important problem of fundamental science. Citation: Kolodiazhnyi, O.I. Keywords: stereochemistry; prebiotic chemistry; origin of life; DNA; RNA; phosphagens; nucleotides; Phosphorus Compounds of Natural Origin: Prebiotic, Stereochemistry, natural phosphonic acids; antibiotics; phosphonopeptides; phosphoramidates Application.