Enhancing Lipid Content in Chlorococcum Littorale Using in Situ Fluorescence Cell Sorting
Total Page:16
File Type:pdf, Size:1020Kb
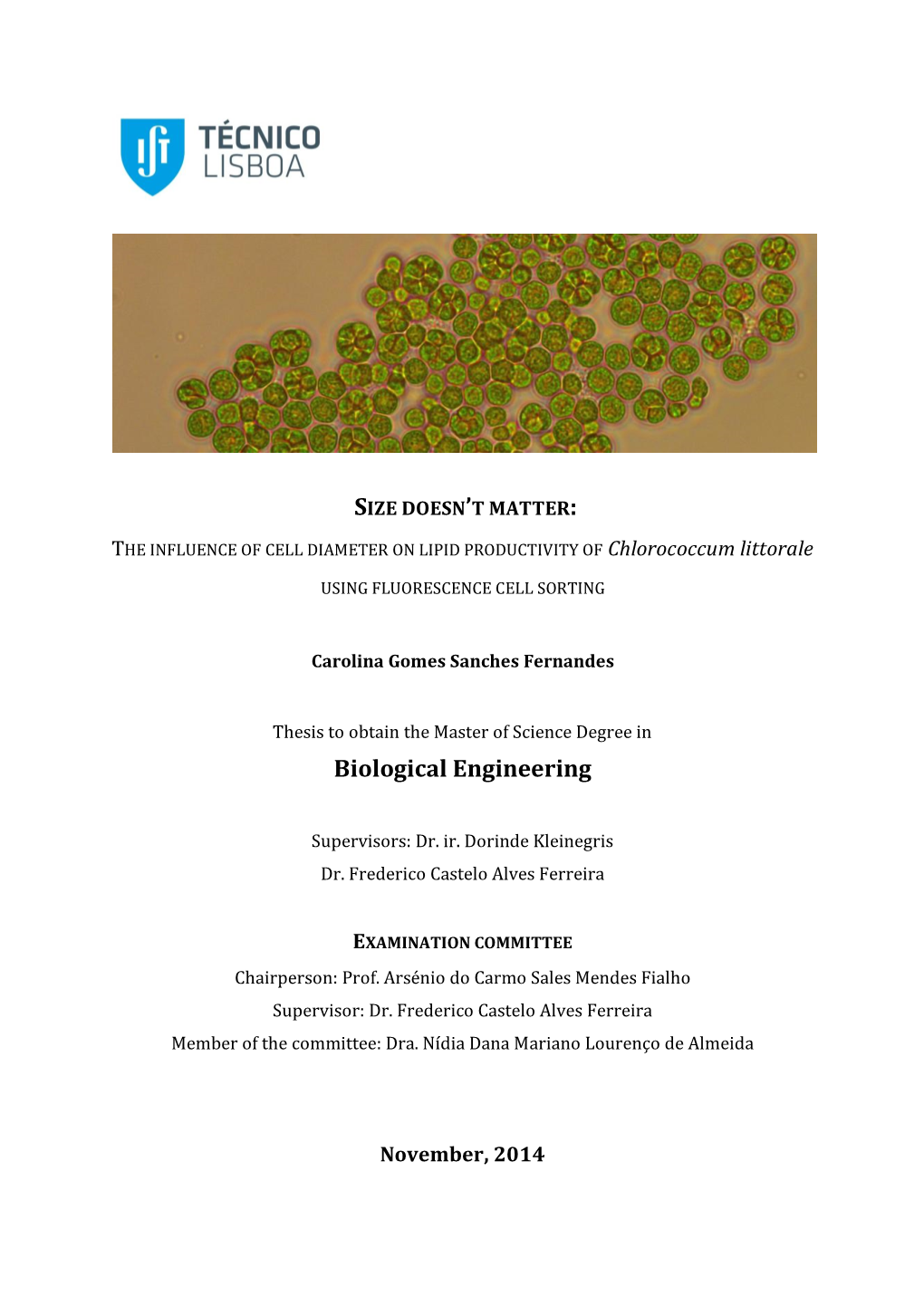
Load more
Recommended publications
-
Emerging Interaction Patterns in the Emiliania Huxleyi-Ehv System
viruses Article Emerging Interaction Patterns in the Emiliania huxleyi-EhV System Eliana Ruiz 1,*, Monique Oosterhof 1,2, Ruth-Anne Sandaa 1, Aud Larsen 1,3 and António Pagarete 1 1 Department of Biology, University of Bergen, Bergen 5006, Norway; [email protected] (R.-A.S.); [email protected] (A.P.) 2 NRL for fish, Shellfish and Crustacean Diseases, Central Veterinary Institute of Wageningen UR, Lelystad 8221 RA, The Nederlands; [email protected] 3 Uni Research Environment, Nygårdsgaten 112, Bergen 5008, Norway; [email protected] * Correspondence: [email protected]; Tel.: +47-5558-8194 Academic Editors: Mathias Middelboe and Corina Brussaard Received: 30 January 2017; Accepted: 16 March 2017; Published: 22 March 2017 Abstract: Viruses are thought to be fundamental in driving microbial diversity in the oceanic planktonic realm. That role and associated emerging infection patterns remain particularly elusive for eukaryotic phytoplankton and their viruses. Here we used a vast number of strains from the model system Emiliania huxleyi/Emiliania huxleyi Virus to quantify parameters such as growth rate (µ), resistance (R), and viral production (Vp) capacities. Algal and viral abundances were monitored by flow cytometry during 72-h incubation experiments. The results pointed out higher viral production capacity in generalist EhV strains, and the virus-host infection network showed a strong co-evolution pattern between E. huxleyi and EhV populations. The existence of a trade-off between resistance and growth capacities was not confirmed. Keywords: Phycodnaviridae; Coccolithovirus; Coccolithophore; Haptophyta; Killing-the-winner; cost of resistance; infectivity trade-offs; algae virus; marine viral ecology; viral-host interactions 1. -
A Taxonomic Reassessment of Chlamydomonas Meslinii (Volvocales, Chlorophyceae) with a Description of Paludistella Gen.Nov
Phytotaxa 432 (1): 065–080 ISSN 1179-3155 (print edition) https://www.mapress.com/j/pt/ PHYTOTAXA Copyright © 2020 Magnolia Press Article ISSN 1179-3163 (online edition) https://doi.org/10.11646/phytotaxa.432.1.6 A taxonomic reassessment of Chlamydomonas meslinii (Volvocales, Chlorophyceae) with a description of Paludistella gen.nov. HANI SUSANTI1,6, MASAKI YOSHIDA2, TAKESHI NAKAYAMA2, TAKASHI NAKADA3,4 & MAKOTO M. WATANABE5 1Life Science Innovation, School of Integrative and Global Major, University of Tsukuba, 1-1-1 Tennodai, Tsukuba, Ibaraki, 305-8577, Japan. 2Faculty of Life and Environmental Sciences, University of Tsukuba, 1-1-1 Tennodai, Tsukuba 305-8577, Japan. 3Institute for Advanced Biosciences, Keio University, Tsuruoka, Yamagata, 997-0052, Japan. 4Systems Biology Program, Graduate School of Media and Governance, Keio University, Fujisawa, Kanagawa, 252-8520, Japan. 5Algae Biomass Energy System Development and Research Center, University of Tsukuba. 6Research Center for Biotechnology, Indonesian Institute of Sciences, Jl. Raya Bogor KM 46 Cibinong West Java, Indonesia. Corresponding author: [email protected] Abstract Chlamydomonas (Volvocales, Chlorophyceae) is a large polyphyletic genus that includes numerous species that should be classified into independent genera. The present study aimed to examine the authentic strain of Chlamydomonas meslinii and related strains based on morphological and molecular data. All the strains possessed an asteroid chloroplast with a central pyrenoid and hemispherical papilla; however, they were different based on cell and stigmata shapes. Molecular phylogenetic analyses based on 18S rDNA, atpB, and psaB indicated that the strains represented a distinct subclade in the clade Chloromonadinia. The secondary structure of ITS-2 supported the separation of the strains into four species. -
2016 National Algal Biofuels Technology Review
National Algal Biofuels Technology Review Bioenergy Technologies Office June 2016 National Algal Biofuels Technology Review U.S. Department of Energy Office of Energy Efficiency and Renewable Energy Bioenergy Technologies Office June 2016 Review Editors: Amanda Barry,1,5 Alexis Wolfe,2 Christine English,3,5 Colleen Ruddick,4 and Devinn Lambert5 2010 National Algal Biofuels Technology Roadmap: eere.energy.gov/bioenergy/pdfs/algal_biofuels_roadmap.pdf A complete list of roadmap and review contributors is available in the appendix. Suggested Citation for this Review: DOE (U.S. Department of Energy). 2016. National Algal Biofuels Technology Review. U.S. Department of Energy, Office of Energy Efficiency and Renewable Energy, Bioenergy Technologies Office. Visit bioenergy.energy.gov for more information. 1 Los Alamos National Laboratory 2 Oak Ridge Institute for Science and Education 3 National Renewable Energy Laboratory 4 BCS, Incorporated 5 Bioenergy Technologies Office This report is being disseminated by the U.S. Department of Energy. As such, the document was prepared in compliance with Section 515 of the Treasury and General Government Appropriations Act for Fiscal Year 2001 (Public Law No. 106-554) and information quality guidelines issued by the Department of Energy. Further, this report could be “influential scientific information” as that term is defined in the Office of Management and Budget’s Information Quality Bulletin for Peer Review (Bulletin). This report has been peer reviewed pursuant to section II.2 of the Bulletin. Cover photo courtesy of Qualitas Health, Inc. BIOENERGY TECHNOLOGIES OFFICE Preface Thank you for your interest in the U.S. Department of Energy (DOE) Bioenergy Technologies Office’s (BETO’s) National Algal Biofuels Technology Review. -
Identification and Characterization of a Symbiotic Alga from Soil Bryophyte for Lipid Profiles
© 2016. Published by The Company of Biologists Ltd | Biology Open (2016) 5, 1317-1323 doi:10.1242/bio.019992 RESEARCH ARTICLE Identification and characterization of a symbiotic alga from soil bryophyte for lipid profiles Jia Feng, Yuning Guo, Xiujuan Zhang, Guihua Wang, Junping Lv, Qi Liu and Shulian Xie* ABSTRACT acid composition profiles for biofuel production. A microalgal strain A symbiotic alga was successfully isolated from the soil moss with high lipid accumulation was obtained fortuitously during research Entodon obtusatus found in the Guandi Mountains, Shanxi Province, on the symbiotic relationships between bryophytes and algae. China, and cultivated under axenic conditions. Morphological observations showed that the symbiotic alga was similar to RESULTS AND DISCUSSION Chlorococcum. Based on phylogenetic analysis of 18S rRNA Morphological features of the symbiotic alga and rbcL genes and internal transcribed spacer (ITS) regions, The bryophyte specimens collected were identified as Entodon Chlorococcum sp. GD was identified as Chlorococcum sphacosum. obtusatus (Entodontaceae) based on macro- and micro- The three data sets were congruent for those aspects of the morphological features (Fig. S1) (Zhu et al., 2002). Voucher topologies that were relatively robust, and differed for those parts of specimens (No. SAS2013018) were deposited in the herbarium of the topologies that were not. This strain was cultured in BG11 medium Shanxi University (SXU). Vegetative cells of the symbiotic algae to test its growth and biodiesel properties. It produced a lipid content appear ellipsoidal to spherical and vary in size in the light microscope of nearly 40%, and achieved biomass concentration of 410 mg l−1 image. Young cell walls are thin and smooth and become thicker with and lipid productivity of 6.76 mg l−1 day−1, with favorable C16:0 growth. -
Freshwater Algae in Britain and Ireland - Bibliography
Freshwater algae in Britain and Ireland - Bibliography Floras, monographs, articles with records and environmental information, together with papers dealing with taxonomic/nomenclatural changes since 2003 (previous update of ‘Coded List’) as well as those helpful for identification purposes. Theses are listed only where available online and include unpublished information. Useful websites are listed at the end of the bibliography. Further links to relevant information (catalogues, websites, photocatalogues) can be found on the site managed by the British Phycological Society (http://www.brphycsoc.org/links.lasso). Abbas A, Godward MBE (1964) Cytology in relation to taxonomy in Chaetophorales. Journal of the Linnean Society, Botany 58: 499–597. Abbott J, Emsley F, Hick T, Stubbins J, Turner WB, West W (1886) Contributions to a fauna and flora of West Yorkshire: algae (exclusive of Diatomaceae). Transactions of the Leeds Naturalists' Club and Scientific Association 1: 69–78, pl.1. Acton E (1909) Coccomyxa subellipsoidea, a new member of the Palmellaceae. Annals of Botany 23: 537–573. Acton E (1916a) On the structure and origin of Cladophora-balls. New Phytologist 15: 1–10. Acton E (1916b) On a new penetrating alga. New Phytologist 15: 97–102. Acton E (1916c) Studies on the nuclear division in desmids. 1. Hyalotheca dissiliens (Smith) Bréb. Annals of Botany 30: 379–382. Adams J (1908) A synopsis of Irish algae, freshwater and marine. Proceedings of the Royal Irish Academy 27B: 11–60. Ahmadjian V (1967) A guide to the algae occurring as lichen symbionts: isolation, culture, cultural physiology and identification. Phycologia 6: 127–166 Allanson BR (1973) The fine structure of the periphyton of Chara sp. -
A Preliminary Survey of the Diversity of Soil Algae And'cyanoprokaryotes'on
Venter, et al. 2015. Published in Australian Journal of Botany. 63:341-352. A preliminary survey of the diversity of soil algae and cyanoprokaryotes on mafic and ultramafic substrates in South Africa , , Arthurita Venter A C, Anatoliy Levanets A, Stefan Siebert A and Nishanta RajakarunaA B AUnit for Environmental Sciences and Management, North-West University, Private Bag X6001, Potchefstroom, 2520, South Africa. BCollege of the Atlantic, 105 Eden Street, Bar Harbor, ME 04609, USA. CCorresponding author. Email: [email protected] Abstract. Despite a large body of work on the serpentine-substrate effect on vascular plants, little work has been undertaken to describe algal communities found on serpentine soils derived from peridotite and other ultramafic rocks. We report a preliminary study describing the occurrence of algae and cyanoprokaryotes on mafic and ultramafic substrates from South Africa. Results suggest that slope and aspect play a key role in species diversity and community composition and, although low pH, nutrients and metal content do not reduce species richness, these edaphic features also influence species composition. Further, typical soil genera such as Leptolyngbya, Microcoleus, Phormidium, Chlamydomonas, Chlorococcum and Hantzschia were found at most sites. Chroococcus sp., Scytonema ocellatum, Nostoc linckia, Chlorotetraedron sp., Hormotilopsis gelatinosa, Klebsormidium flaccidium, Pleurococcus sp. and Tetracystis elliptica were unique to one serpentine site. The preliminary survey provides directions for future research on the serpentine- substrate effect on algal and cyanoprokaryote diversity in South Africa. Additional keywords: algae, cryptogamic ecology, serpentine geoecology, species diversity. Introduction species (Siebert et al. 2002;O’Dell and Rajakaruna 2011) and A range of soils can develop from ultramafic rocks depending on are model settings for the study of plant ecology and evolution climate, time, relief, chemical composition of the parent materials (Harrison and Rajakaruna 2011). -
7 Systematics of the Green Algae
7989_C007.fm Page 123 Monday, June 25, 2007 8:57 PM Systematics of the green 7 algae: conflict of classic and modern approaches Thomas Pröschold and Frederik Leliaert CONTENTS Introduction ....................................................................................................................................124 How are green algae classified? ........................................................................................125 The morphological concept ...............................................................................................125 The ultrastructural concept ................................................................................................125 The molecular concept (phylogenetic concept).................................................................131 Classic versus modern approaches: problems with identification of species and genera.....................................................................................................................134 Taxonomic revision of genera and species using polyphasic approaches....................................139 Polyphasic approaches used for characterization of the genera Oogamochlamys and Lobochlamys....................................................................................140 Delimiting phylogenetic species by a multi-gene approach in Micromonas and Halimeda .....................................................................................................................143 Conclusions ....................................................................................................................................144 -
National Algal Biofuels Technology Roadmap
BIOMASS PROGRAM National Algal Biofuels Technology Roadmap MAY 2010 National Algal Biofuels Technology Roadmap A technology roadmap resulting from the National Algal Biofuels Workshop December 9-10, 2008 College Park, Maryland Workshop and Roadmap sponsored by the U.S. Department of Energy Office of Energy Efficiency and Renewable Energy Office of the Biomass Program Publication Date: May 2010 John Ferrell Valerie Sarisky-Reed Office of Energy Efficiency Office of Energy Efficiency and Renewable Energy and Renewable Energy Office of the Biomass Program Office of the Biomass Program (202)586-5340 (202)586-5340 [email protected] [email protected] Roadmap Editors: Daniel Fishman,1 Rajita Majumdar,1 Joanne Morello,2 Ron Pate,3 and Joyce Yang2 Workshop Organizers: Al Darzins,4 Grant Heffelfinger,3 Ron Pate,3 Leslie Pezzullo,2 Phil Pienkos,4 Kathy Roach,5 Valerie Sarisky-Reed,2 and the Oak Ridge Institute for Science and Education (ORISE) A complete list of workshop participants and roadmap contributors is available in the appendix. Suggested Citation for This Roadmap: U.S. DOE 2010. National Algal Biofuels Technology Roadmap. U.S. Department of Energy, Office of Energy Efficiency and Renewable Energy, Biomass Program. Visit http://biomass.energy.gov for more information 1BCS, Incorporated 2Office of the Biomass Program 3Sandia National Laboratories 4National Renewable Energy Laboratory 5MurphyTate LLC This report is being disseminated by the Department of Energy. As such, the document was prepared in compliance with Section 515 of the Treasury and General Government Appropriations Act for Fiscal Year 2001 (Public Law No. 106-554) and information quality guidelines issued by the Department of energy. -
Diuron Environmental Assessment Report
DIURON ENVIRONMENT ASSESSMENT JULY 2011 ii DIURON CONTENTS ENVIRONMENTAL ASSESSMENT SUMMARY 3 APPENDIX A—CHEMICAL STRUCTURES OF DIURON, ITS METABOLITES AND RELEVANT METABOLLIC PATHWAYS 80 APPENDIX B —REFINEMENT OF AQUATIC TOXICITY ENDPOINT 84 APPENDIX C—RUNOFF VALUES FOR USE IN THE RISK ASSESSMENT 91 APPENDIDIX D - ENVRIONMENTAL FATE 103 1. ABIOTIC DEGRADATION 103 2. METABOLISM 106 3. MOBILITY 122 4. FIELD DISSIPATION STUDIES 127 5. MEASURED FIELD CONCENTRATIONS 142 APPENDIX E - ENVIRONMENTAL EFFECTS 156 1. AVIAN 156 2. AQUATIC TOXICITY 163 3. AQUATIC INVERTEBRATES 174 4. ALGAE, DIATOMS AND AQUATIC PLANTS 188 5. TERRESTRIAL INVERTEBRATES AND SOIL MICROFLORA 214 APPENDIX F - REFERENCES 230 3 ENVIRONMENTAL ASSESSMENT SUMMARY 1 INTRODUCTION The Department of Sustainability, Environment, Water, Population and Communities (DSEWPaC) evaluation of the environmental chemistry and fate is based essentially on the data package provided by Griffin Corporation Australia (DuPont) and Bayer as requested under the APVMA's Chemical Review Program. Additional information is provided by the scientific literature or other international reviews. Since release of the PRF, additional laboratory and monitoring test data have been supplied by DuPont. The assessment of these studies are included in this report. Diuron is a selective herbicide for broadleaf weeds and some annual grasses. It belongs to the urea group of herbicides and is readily absorbed through the root system of plants and less readily through the leaves and stems. Residues in soil are toxic to plants. It is used in many agricultural situations, general weed control in irrigation ditches and drains and in non-agricultural areas (rights-of-way, commercial and industrial areas) and often used in combination with other herbicides such as bromacil and hexazinone. -
(14 May 2020) ISSN 2009-8987 1 Chlorococcum Amblystomatis
!"#$%&'(&%)&*$+(!"#$%&'$(%)$*+,$-.-./$$$$$$$$$$$$$$$$$$$$$$$$$$$$$$$$$$$$$$$$$$$$$$$$$$$$$$$$$$$$011!$-..23'2'4$ ! !"#$%$&$&&'()*(+#,-.$(*./-!"#$%$&'()*+,!*-!./00*1!2$34++*/'5!6$7'+*0'!8!&*49*0!:*+*/+'5! &$(+0)1$20!"!"#$%$&$&&*&3*31! $ !567+$8"99:7+;$,%%+-.*"&%)&'(!&#$*&%(/*"0$.#1(23,34(5'1'&*.6(&70(8'9'%":+'7#(8':&*#+'7#;5$&( <=(0'(,>*-%4(7?(@A4(<BB=CB@D(/&#&-&14(/"*#$)&%( $ <"="$>+9:?+;$EEF,5;E'7#*'("G(F&*-7'(2.-'7.'14(H7-9'*1-#I("G(,%)&*9'4(J&+>'%&14(KLL=C@DA( M&*"4(/"*#$)&%$(@"99:AB"C6:C@:D$EF+9:?+GH+?I#BJ/$ $ K:"C:?$L:9:79+$F,5N;F&*-7'(&70(N79-*"7+'7#&%(2.-'7.'1(E'7#*'4(8':&*#+'7#("G(O-G'(2.-'7.'14( H7-9'*1-#I("G(E"-+>*&4(DLLLCB=P(E"-+>*&4(/"*#$)&%$ $ MN:$O7C+9,$6:A7IC+J7"C$Q":6-%&$&+>%I1#"+&#-1$P#Q#K+RO:9J$S79AJ$+BB:+9:6$7C$JN:$:TA7@@+J+$ /6I."#6'.&(R"*'&%-C,+'*-.&7&$7C$%2.U$+A$C"#$%-V4#$WA$7J$X+A$C"J$+@@"RB+C7:6$O,$+$6:A@97BJ7"C;$7J$ X+A$7CF+?76$+J$JN+J$J7R:#$MN:$I:CHA$+C6$AB:@7:A$6:A7IC+J7"C$X+A$?+J:9$F+?76+J:6$O,$Y7??:$(%2.2D$)4/$ X7JN$O97:S$6:A@97BJ7"CA$"S$JN:$I:CHA$+C6$AB:@7:A$7C$Z:9R+C$+C6$@7J+J7"C$"S$/6I."#6'.&(R"*'&%-C ,+'*-.&7&$C"#$%-V4#$MN:$"97I7C+?$R+J:97+?$X+A$I+JN:9:6$S9"R$:II$R:RO9+C:A$"S$,+>%I1#"+&( :$7.#&#$+$8"B:;$%'V'$+$A+?+R+C6:9;$@"??:@J:6$+J$*766?:A:T$P:??A;$*+AA+@NHA:JJA;$[1W#$ ,+>%I1#"+&(:$7.#&#$+$8"B:;$%'V'$7A$C"X$@"CA76:9:6$J"$O:$+$A,C"C,R$"S$,+>I1#"+&$\1-.]( +&.$%&#$+$(1N+X;$%'.-/#$$ $ MN:$@:??A$"S$Q":6-%&$&+>%I1#"+&#-1$X:9:$7CJ:CA7F:?,$AJH67:6$+C6$S"HC6$J"$O:$@+B+O?:$"S$ 7CJ9+@:??H?+9$A,RO7"A7A$JN+J$7A$S+@H?J+J7F:$9+JN:9$JN+C$"O?7I+J:$(:#I#;$^:9C:,$-.%%_$^:9C:,$'#(&%3$ -.%%/#$^7R$'#(&%#$(-.%)/$S"HC6$Q":6-%&(&+>%I1#"+&#-1$J"$O:$X76:AB9:+6$7C$!"9JN$WR:97@+$+C6$ -
Novel Insights Into the Biotechnological Production Of
Novel Insights into the Biotechnological Production of Haematococcus pluvialis-Derived Astaxanthin: Advances and Key Challenges to Allow Its Industrial Use as Novel Food Ingredient Samuel Jannel, Yanis Caro, Marc Bermudes, Thomas Petit To cite this version: Samuel Jannel, Yanis Caro, Marc Bermudes, Thomas Petit. Novel Insights into the Biotechnological Production of Haematococcus pluvialis-Derived Astaxanthin: Advances and Key Challenges to Allow Its Industrial Use as Novel Food Ingredient. Journal of Marine Science and Engineering, MDPI, 2020, Special Issue ”Diversity and Biotechnological Potential of Marine Microorganisms”, 8 (10), 10.3390/jmse8100789. hal-02995676 HAL Id: hal-02995676 https://hal.univ-reunion.fr/hal-02995676 Submitted on 9 Nov 2020 HAL is a multi-disciplinary open access L’archive ouverte pluridisciplinaire HAL, est archive for the deposit and dissemination of sci- destinée au dépôt et à la diffusion de documents entific research documents, whether they are pub- scientifiques de niveau recherche, publiés ou non, lished or not. The documents may come from émanant des établissements d’enseignement et de teaching and research institutions in France or recherche français ou étrangers, des laboratoires abroad, or from public or private research centers. publics ou privés. Journal of Marine Science and Engineering Review Novel Insights into the Biotechnological Production of Haematococcus pluvialis-Derived Astaxanthin: Advances and Key Challenges to Allow Its Industrial Use as Novel Food Ingredient Samuel Jannel 1,2, Yanis -
Algal Endosymbiosis in Brown Hydra: Host/Symbiont Specificity
J. Cell Sci. 86, 273-286 (1986) 273 Printed in Great Britain © The Company of Biologists Limited 1986 ALGAL ENDOSYMBIOSIS IN BROWN HYDRA: HOST/SYMBIONT SPECIFICITY M. RAHAT AND V. REICH Department of Zoology, The Hebrew University of Jerusalem, Jerusalem 91904, Israel SUMMARY Host/symbiont specificity has been investigated in non-symbiotic and aposymbiotic brown and green hydra infected with various free-living and symbiotic species and strains of Chlorella and Chlorococcum. Morphology and infrastructure of the symbioses obtained have been compared. Aposymbiotic Swiss Hydra viridis and Japanese H. magnipapillata served as controls. In two strains of H. attenuata stable hereditary symbioses were obtained with Chlorococcum isolated from H. magnipapillata. In one strain of H. vulgaris, in H, oligactis and in aposymbiotic H. viridis chlorococci persisted for more than a week. Eight species of free-living Chlorococcum, 10 symbiotic and 10 free-living strains of Chlorella disappeared from the brown hydra within 1-2 days. In H. magnipapillata there was a graded distribution of chlorococci along the polyps. In hypostomal cells there were >30 algae/cell while in endodermal cells of the mid-section or peduncle <10 algae/cell were found. In H. attenuata the algal distribution was irregular, there were up to five chlorocci/cell, and up to 20 cells/hydra hosted algae. In the dark most cells of Chlorococcum disappeared from H. magnipapillata and aposymbiotic hydra were obtained. Chlorococcum is thus an obligate phototroph, and host-dependent hetero- trophy is not required for the preservation of a symbiosis. The few chlorococci that survived in the dark seem to belong to a less-demanding physiological strain.