Secondary Clay Formation, Element Leaching, and M
Total Page:16
File Type:pdf, Size:1020Kb
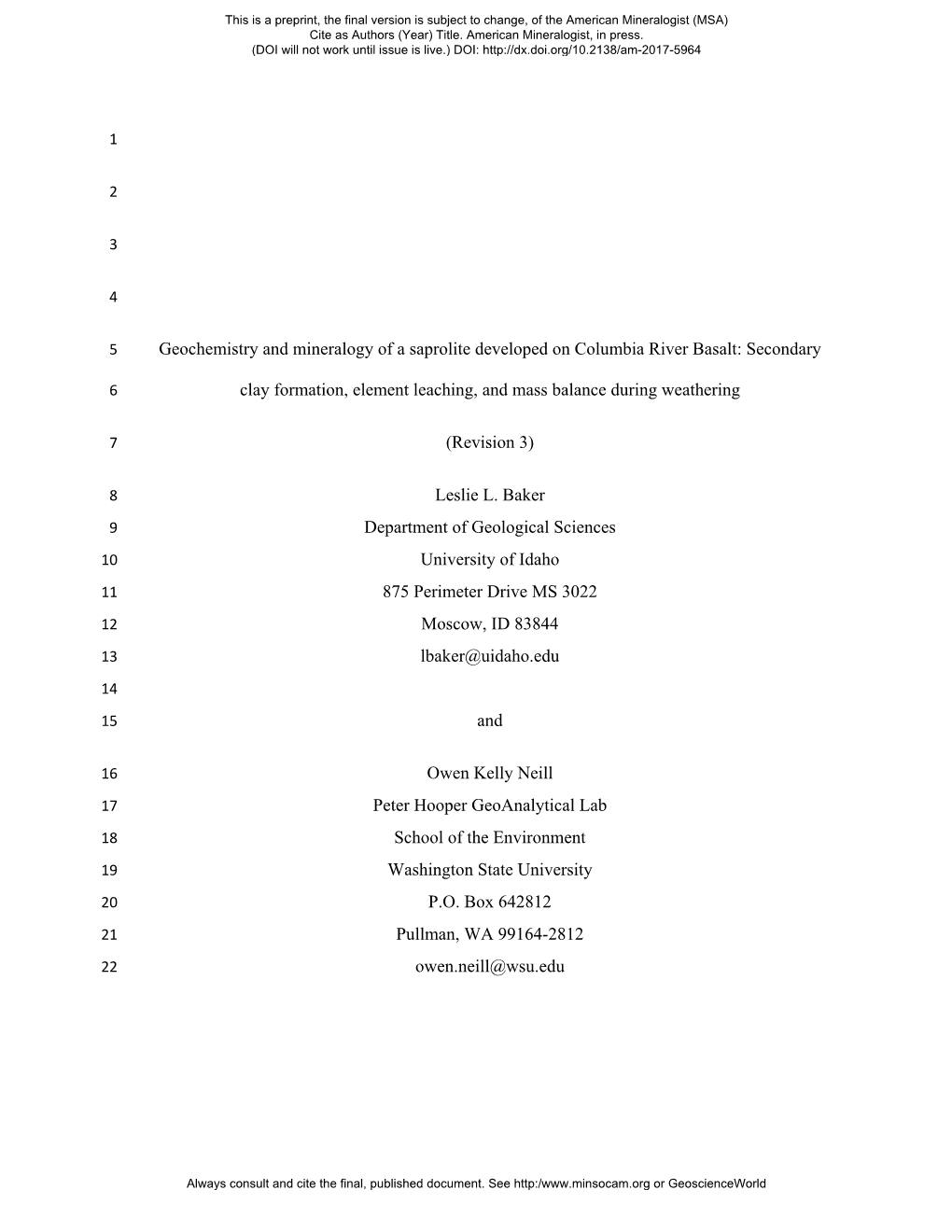
Load more
Recommended publications
-
A Review of Feldspar Alteration and Its Geological Significance in Sedimentary Basins from Shallow Aquifers to Deep Hydrocarbon
Originally published as: Yuan, G., Cao, Y., Schulz, H.-M., Hao, F., Gluyas, J., Liu, K., Yang, T., Wang, Y., Xi, K., Li, F. (2019): A review of feldspar alteration and its geological significance in sedimentary basins: From shallow aquifers to deep hydrocarbon reservoirs. - Earth-Science Reviews, 191, pp. 114—140. DOI: http://doi.org/10.1016/j.earscirev.2019.02.004 Earth-Science Reviews 191 (2019) 114–140 Contents lists available at ScienceDirect Earth-Science Reviews journal homepage: www.elsevier.com/locate/earscirev A review of feldspar alteration and its geological significance in sedimentary basins: From shallow aquifers to deep hydrocarbon reservoirs T ⁎ ⁎ Guanghui Yuana,b, , Yingchang Caoa,b, , Hans-Martin Schulzc, Fang Haoa, Jon Gluyasd, Keyu Liua, Tian Yanga, Yanzhong Wanga, Kelai Xia, Fulai Lia a Key laboratory of Deep Oil and Gas, School of Geosciences, China University of Petroleum, Qingdao, Shandong 266580, China b Laboratory for Marine Mineral Resources, Qingdao National Laboratory for Marine Science and Technology, Qingdao, Shandong 266071, China c GFZ German Research Centre for Geosciences, Section 4.3, Organic Geochemistry, Telegrafenberg, D-14473 Potsdam, Germany d Department of Earth Sciences, Durham University, Durham DH1 3LE, UK ARTICLE INFO ABSTRACT Keywords: The feldspar group is one of the most common types of minerals in the earth's crust. Feldspar alteration (in- Feldspar alteration cluding the whole processes of feldspar dissolution, transfer of released solutes, and secondary mineral pre- Dissolution mechanisms cipitation) is ubiquitous and important in fields including resources and environmental sciences. This paper Rate law provides a critical review of feldspar alteration and its geological significance in shallow aquifers to deep hy- Organic-inorganic interaction drocarbon reservoirs, as assessed from peer-reviewed paper in the literature. -
Mineralogy of a Layered Gabbro Deformed During Magmatic
American Mineralogist, Volume 74, pages 101-112, 1989 Mineralogy of a layered gabbro deformedduring magmatic crystallizationo western Sierra Nevada foothills" California Rorrnr K. SpmNcrn Departmentof Geology,Brandon University, Brandon, Manitoba R7A 6A9, Canada ABSTRACT In the 162 Ma Pine Hill intrusive complex of northern California, a synformal, layered pyroxenite-gabbro-dioritebody, the crystallization sequenceand compositional variation of cumulus and intercumulus minerals indicate that magmatic crystallization was char- acteized by a high initial oxygenfugacity, log,ofo,in the rangeof -4 to -9, which allowed the early precipitation of abundant titanomagnetite; the oxygen fugacity decreasedless than two log units with crystallizalion. The magmatic crystallization sequenceis ol + aug, ol + aug * mt, ol + aug + mt + pl, ol + aug + mt + pl + opx(invertedfrom pig) and aug + mt + pl + opx (inverted from pig) * qz. Pigeonite crystallization is evidenced by 001 exsolution lamellae in orthopyroxene of Enrr. Superimposedupon this sequenceare intercumulus minerals, which crystallized from magma trapped within cumulates, and biotite and calcic amphibole, which largely formed from a subsolidusreaction of a residual hydrous fluid phasewith primary minerals. Mineral compositional rangesare plagioclase, Ann, to Anrr; olivine, Fo, to Fooo;augite, CanrMgrFento CaorMg.rFerr;Ca-poor pyroxene, Ca,Mgr"Fe' to Ca,MgorFeon;Mgl(Mg * Fe * Mn) of calcic amphibole, 0.74 to 0.48; and Me/(Me * Fe * Mn) of biotite, 0.78 to 0.45. Prior to complete solidification (>900/o crystallized), deformation of the complex coincided with an abrupt decreasein Mg/(Mg * Fe) values for the mafic silicates. This compositional discontinuity is attributed to a decreasein oxygen fugacity of the crystallizing magma causedby an exchangebetween the fluid phaseof adjacentcountry rock and the fluid phaseof the magma during deformation. -
Primary Igneous Analcime: the Colima Minettes
American Mineralogist, Volume 74, pages216-223, 1989 Primary igneous analcime: The Colima minettes Jlnrns F. Lurrn Department of Earth and Planetary Sciences,Washington University, St. Louis, Missouri 63 130, U.S.A. T. Kunrrs Kvsnn Department of Geological Sciences,University of Saskatchewan,Saskatoon, Saskatchewan S7N 0W0, Canada Ansrnrcr Major-element compositions, cell constants, and oxygen- and hydrogen-isotopecom- positions are presentedfor six analcimes from differing geologicenvironments, including proposed primary @-type) analcime microphenocrysts from a late Quaternary minette lava near Colima, Mexico. The Colima analcimehas X.,.,",: 0.684, ao: 13.712A, and one of the lowest D'sOvalues yet recorded for analcime (+9.28m).The major difficulty in identifuing primary @-type) igrreousanalcime is distinguishing it from analcime formed by ion-exchangeconversion of leucite (L-type analcime) or other precursor minerals. Pet- rographic criteria are shown to be unreliable in discriminating P and L analcimes,although the higher KrO and Rb contents and D'8Ovalues of L-type crystals may be diagnostic. P- and L-type analcimescan be distinguishedfrom classichydrothermal varieties (H-type) by the lower Fe contents of the latter. H-type analcimes,however, can have 6t8Ovalues as low as 8.9Vmand cannot be distinguished from P-type analcimes by this criterion. Analcimes formed from volcanic glass or zeolite precursorsin saline, alkaline lakes (S- type) or metamorphic sequences(M-type) can be distinguished from other varieties by their higher silica contents, smaller cell constants,and higher d'8O values of > + 17.7Vm. For all types of analcime, d'8O of the channel water does not correlate with 6180of the framework oxygen. -
Petrography Edward F
Chapter 4 Petrography Edward F. Stoddard A petrographic study was taken in order to help determine the sources of lithic artifacts found at archaeological sites on Fort Bragg. In the first phase of the study, known and suspected archaeological quarry sites in the central Piedmont of North Carolina were visited. From each quarry, hand specimens were collected and petrographic thin sections were examined in an attempt to establish a basis for distinguishing among the quarries. If material from each quarry was sufficiently distinctive, then quarry sources could potentially be matched with Fort Bragg lithic artifacts. Seventy-one samples from 12 quarry zones were examined (Table 4.1). Thirty- one of these samples are from five quarry zones in the Uwharrie Mountains region; 20 of these were collected and described previously by Daniel and Butler (1996). Forty specimens were collected from seven additional quarry zones in Chatham, Durham, Person, Orange, and Cumberland Counties. All quarries are within the Carolina Terrane, except the Cumberland County quarry, which occurs in younger sedimentary material derived primarily from Carolina Terrane outcrops. Rocks include both metavolcanic and metasedimentary types. Compositionally, most metavolcanic rocks are dacitic and include flows, tuffs, breccias, and porphyries. Metasedimentary rocks are metamudstone and fine metasandstone. The Uwharrie quarries are divided into five zones: Eastern, Western, Southern, Asheboro, and Southeastern. The divisions are based primarily on macroscopic petrography and follow the results of Daniel and Butler (1996); the Uwharries Southeastern zone was added in this study. Each of the Uwharrie quarry zones represents three to six individual quarries in relatively close proximity. Rock specimens are all various felsic metavolcanic rocks, but zones may be distinguished based upon mineralogy and texture. -
Mineralogical Characteristics of Hydrothermally-Altered Andesite in Kalirejo Village and the Surrounding Areas, Indonesia
Journal of Applied Geology, vol. 3(2), 2018, pp. 99–108 DOI: http://dx.doi.org/10.22146/jag.48598 Mineralogical Characteristics of Hydrothermally-altered Andesite in Kalirejo Village and The Surrounding Areas, Indonesia Diyan Aditya Putra Pratama, I Gde Budi Indrawan,* and I Wayan Warmada Department of Geological Engineering, Faculty of Engineering, Gadjah Mada University, Yogyakarta, Indonesia ABSTRACT. Type and intensity of hydrothermal alterations affect rock engineering prop- erties and slope stability. Identification of mineralogical characteristics of rocks is essen- tial in determination of rock slope failure mechanism in a hydrothermal alteration zone. This research was conducted to identify mineralogical characteristics of hydrothermally- altered andesite in Kalirejo Village and surrounding areas, Indonesia. The research was conducted by field observation and laboratory analyses involving petrographic and X-ray Powder Diffraction (XRD) analyses. The results showed that the research area was domi- nated by argillic alteration type and high alteration intensity implying high susceptibility to slope failures. Keywords: Hydrotermal alteration · Mineralogical characteristics · Andesite · Kalirejo Vil- lage · Indonesia. 1I NTRODUCTION more detailed zonation of areas susceptible to The research area was located in Kalirejo Vil- slope failures. lage and the surroundings. It was adminis- Stability of rock slopes in tectonically active tratively located in Kulon Progo Regency (Yo- and tropical regions is controlled by several fac- gyakarta Special Province) and Purworejo Re- tors, such as slope geometry, material proper- gency (Central Java Province), Indonesia. The ties, groundwater, surface cover, and active fac- regional geological map produced by Rahardjo tors involving earthquake and rainfall. Previ- et al. (1995) indicated that the research area ous publications (e.g., Bell, 2007; Pola et al., was composed of intrusive igneous rock group 2012) have shown that hydrothermal alteration of andesite. -
Chapter 5 Geochemical Weathering
1 Chapter 6 Weathering Chapter 5 Geochemical Weathering Weathering of landscapes involves an array of mechanical and geochemical agents that conspire to alter primary geological formations to sediments and solutes. Geochemical weathering is driven by water. In soils, water is the limiting factor for the activity of aerobic bacteria that degrade organics and produce CO2 at partial pressures orders of magnitude higher than in the atmosphere. Soil CO 2 is hydrated by soil water, providing carbonic acid for the dissolution of primary carbonate and silicate minerals. Water also hosts the bacterial oxidation of sulfides, ferrous iron and other redox reactions in the weathering of minerals. Finally, it is water, with its polar character and capacity to hydrate and hydroxylate that facilitates dissolution of primary mineral phases and transport of solutes in groundwater. Primary phases include high temperature minerals formed by magmatic and metamorphic processes ― the aluminosilicate granites and gneisses that make up shield terrains of continental cratons, and ferromagnesian silicates like basalts that dominate in volcanic belts. The kinetics of silicate weathering are slow, with the formation of secondary clays ― hydrated aluminosilcate minerals with high capacity for cation exchange and sorption. Weathering of carbonate rocks proceeds more rapidly than weathering of silicate rocks. These are dissolution reactions, generating porosity and accelerating water movement. Carbonate minerals are ubiquitous, not just as limestone and dolomite formations, but as secondary phases filling fractures in silicate rocks, and as cements and crusts in soils. In near-surface environments found in unsaturated soils and fractures, CO 2 and O 2 consumed by reaction are continually replenished by diffusion into water from the soil air or open atmosphere. -
The Rock Cycle Journey and Mineral Deposits
The Rock Cycle Journey and Mineral Deposits Overview Students will use observation, inference, and data collection skill to discover that the rock cycle, like the water cycle, has various phases and does not necessarily move linearly through those phases Students will make the connection between rock type, mineral deposit type and processes as they travel through the rock cycle. Source: The original idea for this lesson comes from a lesson by DLESE Mountain Building Teaching Box. It has been adapted for Saskatchewan schools by the SMA. Duration: one – two classes Materials: Rock Cycle Journey Paper dice Rock Cycle Journey Station and Mineral Deposits cards Student Data Table Mineral Deposits Chart Student Rock Cycle Journey Summary Student Cartoon Sheet Saskatchewan Rock Kit (optional): see Resources Keepers of the Earth: “Tunka-shila, Grandfather Rock”; Learning Outcomes and Indicators “Old Man Coyote and the Rock” Grade 7: Earth’s Crust and Resources EC7.1 analyse societal and environmental impacts of Prior Knowledge: historical and current catastrophic geological events, Before attempting these activities students should have and scientific understanding of movements and forces some understanding of the following: within Earth’s crust. Metamorphic, Sedimentary and Igneous rocks b. Provide examples of past theories and ideas, including cultural mythology, that explain geological phenomena such as volcanic activity, earthquakes, and mountain Note to Teachers: building. (Extension 1.) In this activity, rock cycle phases (igneous, sedimentary and metamorphic), processes, such as melting, cooling EC7.2 Identify locations and processes used to extract (crystallization), weathering, erosion, burial, Earth’s geological resources and examine the impacts of deformation, heating, and information about those locations and processes on society and the Saskatchewan mineral deposits are located at 11 environment. -
A Field, Petrographic, and Geochemical Study of Gabbros and Related Rocks from the Sandy Islands Gabbro Complex, Wollaston Domain 1
A Field, Petrographic, and Geochemical Study of Gabbros and Related Rocks from the Sandy Islands Gabbro Complex, Wollaston Domain 1 C. Madore 2 and I.R. Annesley2 Madore, C. and Annesley, I.A. (1994): A field, petrographic, and geochemical study of gabbros and related rocks from the Sandy Islands Gabbro Complex, Wollaston Domain: in Summary of Investigations 1994, Saskatchewan Geological Survey, Sask. Energy Mines, Misc. Rep. 94-4. The Sandy Islands Gabbro Complex is well exposed on Early Proterozoic(?) age). The main belt of Wollaston a series of northeast-trending islands in the west-central Group metasediments is composed of graphitic pelitic part of Wollaston lake near the eastern edge of the gneiss, metamorphosed iron formation, pelitic gneiss, Athabasca Basin (Figure 1 ). It comprises an intermedi calc-silicate gneiss, psammopelitic gneiss, psammitic ate to mafic southeastern component and a felsic to in gneiss, metaquartzite, and amphibolites. The Wollaston termediate northwestern component (shown as an Group rocks are complexly deformed, polymetamor approximate contact on Figure 2, after Chandler, 1978). phosed, and rest unconformably (and tectonically) on re worked, antiformal Archean granitoid gneisses. The Preliminary mapping and sampling was undertaken in Wollaston Group metasediments are intruded by meta 1993 for petrological and geochronological purposes as gabbros, porphyritic granites (1815 Ma), and pegmatites part of an industry-funded geological investigation. Geo (Madore and Annesley, 1993). The precise age of the chemical results indicated possible potential for Au and Cu mineralization. Further work was undertaken in 1994 to investigate the primary (igneous) and secondary (metamorphic) characteristics, the petrochemistry, the tectonic environment, and the economic potential of the Sandy Islands Gabbro Complex. -
The Diorites of Yzerfontein, Darling, Cape Province
T".dE DIORITES OF yzEP.FOi'l"'TEIN, DARLING, CAPE PROVINCE By s. MASKE, Gubmi tted in partial fulfillment of the requirements for the degree of Master of Science in the Faculty of Science, Uni versity of Stellenbosch ·\ ·November 1951 Stellenbosch University https://scholar.sun.ac.za THE DIORITES OF YZERFONTEIN, DARLING, CAPE PROVINCE By S. MASKE, B.Sc. (Submitted November, 1951) ABSTRACT The recent suggestion of the existence of a basaltic magma in the South-west Cape Province shortly before the intrusion of the Cape granites is corroborated by the occurrence of a gabbroic body of pre-granite age at Yzerfontein. A detailed study of the primary rhythmic banding and igneous lamination of this body indicates its original form as being sheet-like or Iaccolithic, and the differentiation appears to have been due to a combination of fractional crystallisation and gravitative crystal settling on to a sub-horizontal floor. The crystal settling process was probably later arrested by the viscosi ty of the rest magma, due to its enrichment in potash and alumina. The Yzerfontein diorites proper represent a hybrid product resulting from the mi xi ng, in depth, of the marginal contaminated facies of the Darling granite with gabbroic material. The present uniformity of the diorite is ascribed to the emplacement of the hybrid magma to a higher leve l. Gabbroic xenocrysts are widely distributed in the diorites, these si ngle crystals almost invariably being surrounded by rims of material later in the reaction series. Amongst the mafic minerals a uniform orientation relation is found to exist between the cores and rims of such reaction products. -
Chemical Geology Mineral Inclusion Assemblage and Detrital Zircon
Chemical Geology 477 (2018) 151–160 Contents lists available at ScienceDirect Chemical Geology journal homepage: www.elsevier.com/locate/chemgeo Mineral inclusion assemblage and detrital zircon provenance T ⁎ Elizabeth A. Bell , Patrick Boehnke1,2, T. Mark Harrison, Matthew M. Wielicki3 Dept. of Earth, Planetary, and Space Sciences, University of California at Los Angeles, 595 Charles E. Young Dr. East/3806 Geology Building, Los Angeles, CA 90095, United States ARTICLE INFO ABSTRACT Editor: Catherine Chauvel Mineral inclusions are common in magmatic zircon and a potentially rich source of petrologic information. fi Keywords: Controls on the relative proportions of inclusion phases, speci cally early-crystallizing minerals such as apatite Hadean and late-crystallizing phases such as quartz, K-feldspar, and muscovite, have not been systematically studied. For Archean instance, apatite dominates many magmatic zircon inclusion suites, and selective replacement of apatite over Zircon other phases has been proposed as a mechanism for generating apatite-poor inclusion assemblages in detrital Mineral inclusion zircons. However, the extent to which apatite inclusion abundance is influenced by source rock composition has Detrital zircon not been established. The preservation of characteristic minerals in granite series, such as differences in mag- netite and ilmenite abundances due to varying redox, have also not been systematically explored as inclusion phases in zircon. We surveyed zircon inclusion assemblages in Phanerozoic granitoids of a range of compositions and found a broadly inverse relationship between the presence of apatite in the inclusion suite and whole-rock SiO2 content. Selective loss of apatite is evident from deficits in apatite content among inclusions in contact with cracks in both detrital zircons and some granitoid zircons with independent evidence for fluid ingress (i.e., secondary phases filling open cracks). -
Electron Microprobe Investigation of Primary Minerals in Basalts from the West Philippine Sea Basin (Ocean Drilling Program Leg 195, Site 1201)1
Shinohara, M., Salisbury, M.H., and Richter, C. (Eds.) Proceedings of the Ocean Drilling Program, Scientific Results Volume 195 8. DATA REPORT: ELECTRON MICROPROBE INVESTIGATION OF PRIMARY MINERALS IN BASALTS FROM THE WEST PHILIPPINE SEA BASIN (OCEAN DRILLING PROGRAM LEG 195, SITE 1201)1 Massimo D’Antonio2 and Mette B. Kristensen3 ABSTRACT 1D’Antonio, M., and Kristensen, M.B., The basement cored at Site 1201 (west Philippine Basin) during 2005. Data report: Electron Ocean Drilling Program Leg 195 consists of a 91-m-thick sequence of microprobe investigation of primary basalts, mostly pillow lavas and perhaps one sheet lava flow, with a few minerals in basalts from the west intercalations of hyaloclastite and interpillow sedimentary material. Philippine Sea Basin (Ocean Drilling Program Leg 195, Site 1201). In Hydrothermal alteration pervasively affected the basalt sequence, giv- Shinohara, M., Salisbury, M.H., and ing rise to a variety of secondary minerals such as K-Fe-Mg-clay miner- Richter, C. (Eds.), Proc. ODP, Sci. als, oxyhydroxides and clay minerals mixtures, natrolite group zeolites, Results, 195, 1–24 [Online]. Available analcite, alkali feldspar, and carbonate. The primary minerals of pillow from World Wide Web: <http:// and sheet basalts that survived the intense hydrothermal alteration www-odp.tamu.edu/publications/ 195_SR/VOLUME/CHAPTERS/ were investigated by electron microprobe with the aim of characteriz- 108.PDF>. [Cited YYYY-MM-DD] ing their chemical composition and variability. The primary minerals 2Dipartimento di Scienze della Terra, are mostly plagioclase, ranging in composition from bytownite through Università degli Studi di Napoli labradorite to andesine, chromian-magnesian-diopside, and spinels, “Federico II,” Largo S. -
The Phosphorus Concentration of Common Rocks—A Potential Driver of Ecosystem P Status
Plant Soil DOI 10.1007/s11104-012-1490-2 REGULAR ARTICLE The phosphorus concentration of common rocks—a potential driver of ecosystem P status Stephen Porder & Sohini Ramachandran Received: 21 June 2012 /Accepted: 3 October 2012 # Springer Science+Business Media Dordrecht 2012 Abstract (several alkali basalts). Median P was significantly Background Soil phosphorus (P) availability can be an lower in common silica-rich rocks (e.g. granite - important regulator of ecosystem processes. Changes in 436 ppm) and higher in common iron-rich rocks (e.g. P availability over time have long been studied, but the andesite - 1,000 ppm). In sedimentary rocks, which P concentration of soil parent materials—which deter- make up 70 % of the ice-free land surface, median P mines ecosystem P concentration at the onset of soil was highest in mudstone (1,135 ppm) and decreased formation—have never been systematically explored. with increasing grainsize (siltstone-698 ppm, Here we ask two questions: 1) how does P concentration sandstone-500 ppm). Where soil P and parent material vary among soil parent materials? and 2) under what P were measured in the same site, parent material P range of conditions do those differences influence soil P explained 42 % of the variance in total soil P (n062), concentration? and explanatory power was higher for sites with sim- Methods We used the Earthchem webportal to com- ilar climate. pile the P concentration of 263,539 rocks. We then Conclusion The variation in P concentration among gathered data from 62 sites (MAT ranging from 200– common rock types is on a comparable scale to the 5,000 mmyr-1 and soil age from 0.3–4,100 ky) and changes in total P, and several P pools, over long-term assessed the correlation between rock and soil P soil development.