Cuttlefish Early Development and Behaviour Under Future High CO2 Conditions
Total Page:16
File Type:pdf, Size:1020Kb
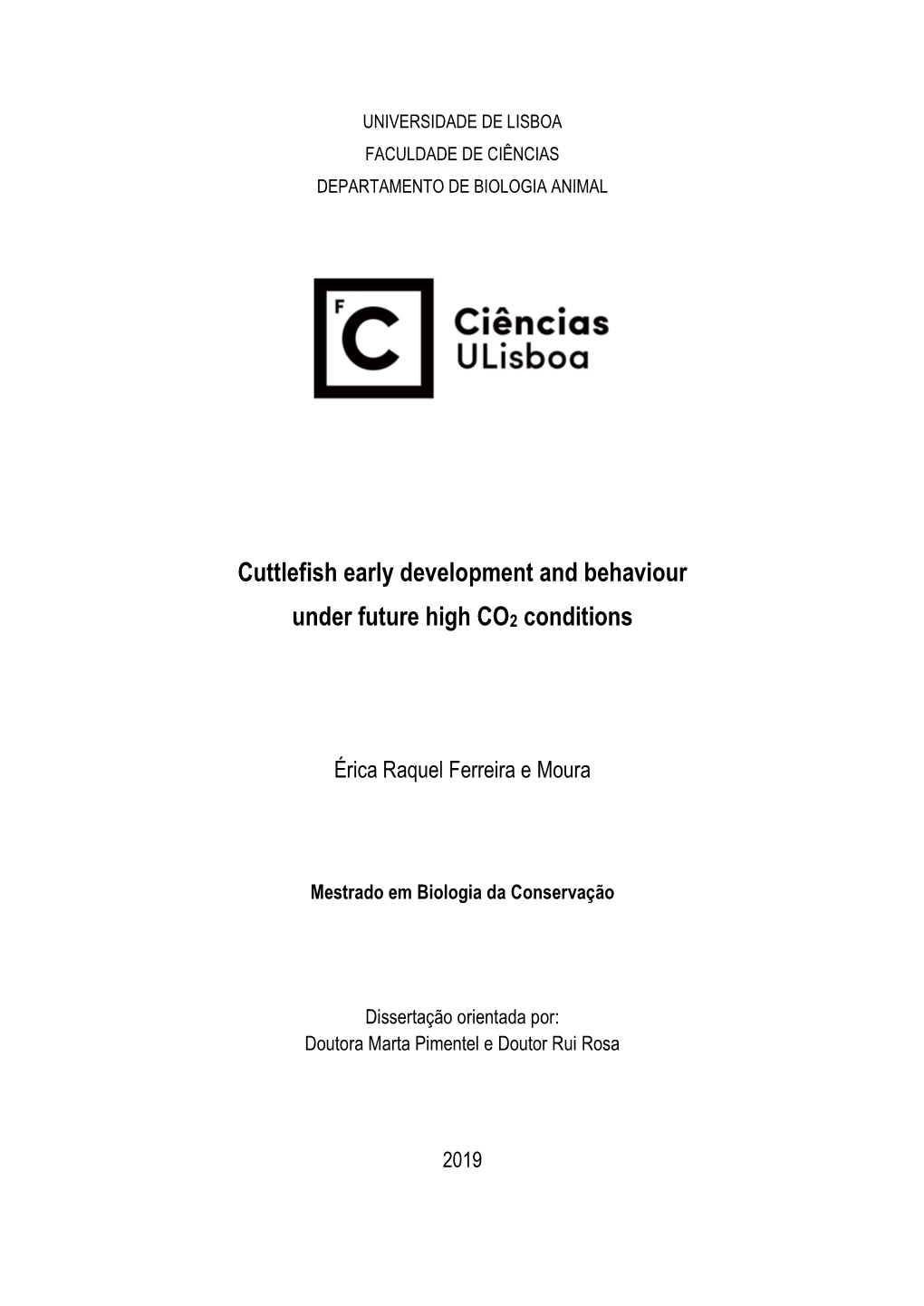
Load more
Recommended publications
-
Annual Report 2014
AR 14 ARC CENTRE OF EXCELLENCE FOR CORAL REEF STUDIES ANNUAL REPORT 2014 Contents 2 Vision 2 Aims 2 Overview 4 Director’s Report 6 2014 Highlights 7 Global Research Reach 8 Chief Investigator Profile: Associate Professor Sophie Dove 10 Graduate Profile: Georgina Gurney 11 Research Program 1: People and Ecosystems 17 Research Program 2: Ecosystem Dynamics: Past Present and Future 23 Research Program 3: Responding to a Changing World 29 Article: Single Species May be Key to Reef Health 30 Knowledge Transfer 32 Graduate and Early Career Training At the ARC Centre of Excellence for Coral Reef Studies we acknowledge the Australian Aboriginal and Torres Strait Islander peoples of this nation. We acknowledge the Traditional Owners of the lands where we conduct our business. We pay our respects to ancestors and Elders, past, present and future. Cover photo by Raphael Williams Photo by Stefano Montanari 41 National and International Linkages 44 Article: Effects of Changing Tastes in China Extend Abroad 46 Media and Public Outreach 49 National Research Priority Case Study: The Great Barrier Reef 51 Article: Plan to Protect Great Barrier Reef Under Fire 52 Publications 62 Recognition of Excellence by Centre Members 64 Governance 66 Leader Profile: Professor Katrina Brown 67 Membership 69 Financial Statement 70 Financial Outlook 71 Key Performance Indicators 75 Acknowledgements AR 14 Photo by Steve Lindfield Vision Aims Overview 2 CORAL REEF STUDIES Leading the global research effort in the provision of scientific knowledge necessary for sustaining the ecosystem goods and services of the world’s coral reefs during a period of unprecedented environmental change. -
Feeding, Anatomy and Digestive Enzymes of False Limpet Siphonaria Guamensis
World Journal of Fish and Marine Sciences 5 (1): 104-109, 2013 ISSN 2078-4589 © IDOSI Publications, 2013 DOI: 10.5829/idosi.wjfms.2013.05.01.66144 Feeding, Anatomy and Digestive Enzymes of False Limpet Siphonaria guamensis K.V.R. Murty, A. Shameem and K. Umadevi Department of Marine Living Resources Andhra University, Visakhapatnam 530 003, A.P., India Abstract: Very little information has been available in the literature on the feeding habits, anatomy and histology of digestive system of siphonariid limpets. The present study revealed Siphonaria guamensis feeds on the crustose red alga Hildenbrandia prototypus browsing on the rocks by rasping action of radula. The anatomy of digestive system of Siphonaria guamensis is similar with that of the other siphonariid limpets but the length of gut and colon are shorter than the patellogastropod limpets like Cellana radiata, patella vulgata, Fissurella barbadensis and species of Acmaea. The salivary glands are the main source of the enzyme system of Siphonaria guamensis. They contained enzymes which can act on carbohydrates, proteins and polysaccharides. The enzyme which can act on proteins was found only in salivary glands and not detected in any other part of the digestive system. No lypolytic activity was seen in any part of the digestive system of the animal. Key words: False Limpet Feeding Anatomy Digestive Enzymes INTRODUCTION tridentatum and C. minimum, where he described the morphology and histology of the digestive system at Little work has been done on the feeding, digestion length. anatomy and histology of the digestive organs of limpets Very little information has been available in the with an exception of patella vulgata (Davies and Fleure literature on the feeding methods, anatomy and histology [1], Graham [2], Stone and Morton [3], Fretter and Graham of the digestive system of siphonariid limpets. -
Zoology Department, University of Cape Town. Patella Vulgata and P. Aspersa Are Attacked by Haematopus Ostralegus, P. Aspersa Be
THE RESPONSES OF SOUTH AFRICAN PATELLID LIMPETS TO INVERTEBRATE PREDATORS G M BRANCH Zoology Department, University of Cape Town. Accepted: February 1978 ABSTRACT The starfISh Marthasterias glacialis is a generalized predator, feeding particularly on Choromytillis meridiOflalis, but also on several limpets, notably Patella longicosta. T1Iais d"bia (Gastropoda) feeds mainly on barnacles, mussels, and Patella gran"laris. The gastropods BumUJH!na delalandii and B. cincta are principally scavengers, feeding on damaged or dead animals. The responses of Patella spp. to these predators are described. P. gran"laris. P. concolor. P. compressa and P. miniata all retreat rapidly on contact. Small P. grallQtina and P. oculus respond similarly, but larger specimens react aggressively, smashing their shells downwards and often damaging the predator. The territorial species (P.longicosta. P. coch/ear and P. tablilaris) all retreat to their scan and remain clamped there. P. argenvillei and P. tablilaris are usually unresponsive, possibly because they are too large to fall prey. Cellana capensis rolls its mantle upwards to cover the shell, preventing predators from attaching. The responses and their effectiveness are discussed in relation to other behavioural patterns displayed by limpets. There is no correlation between the intensity of a prey's response to a predator and the degree of contact . ) 0 between the two in the field. 1 0 2 d e INTRODUCTION t a d A variety of limpet predators has been recorded. Several birds attack limpets by knocking ( r them off the rock, and either picking out the flesh or consuming the whole limpet and e h s regurgitating the shell: oyster catchers (Haemlltopus spp.), various gulls (LaTUS spp.) and the i l b u sheathbill (Chionus alba) (Test 1945; Feare 1971; Walker 1972). -
Where Can We Find Limpets?
We See Sea Shells by the Seashore Where can we find limpets? Isabella and Evangeline Burns Introduction On holidays our family often goes to the town of Ulladulla on the New South Wales south coast. When we do we always visit the rock pools and rock platform. We always look forward to these trips. We look for pretty starfish and gooey limpets and dead, dried-out blue bottles that pop when we step on them. We enjoy these expeditions so much that we wanted to learn more about some of the animals that live on the rock platform. Many animals live on the rock platform. There are starfish, sea snails and crabs. We decided to look at limpets because they have a pretty shell with many different coloured stripes and the rock platform is covered in them. Also, limpets are well known for being very strong and very difficult to pull off the rocks. This was an animal worth knowing about. Aim We wanted to find out where on the rock platform limpets live. Do they live in the wet or the dry areas of the rock platform? 2 Background Research The animal we are looking at is called the Variegated Limpet. Its scientific name is Cellana tramoserica. It is found on the south-eastern coast of Australia from Queensland to South Australia. Source: www.mesa.edu.au Limpets are gastropods. This means that that they are like snails. They have oval shells shaped like a tent to protect their soft squishy body. The soft squishy part is called their foot! They also have gills to breathe underwater. -
Gastropoda, Mollusca)
bioRxiv preprint doi: https://doi.org/10.1101/087254; this version posted November 11, 2016. The copyright holder for this preprint (which was not certified by peer review) is the author/funder, who has granted bioRxiv a license to display the preprint in perpetuity. It is made available under aCC-BY-NC-ND 4.0 International license. An annotated draft genome for Radix auricularia (Gastropoda, Mollusca) Tilman Schell 1,2 *, Barbara Feldmeyer 2, Hanno Schmidt 2, Bastian Greshake 3, Oliver Tills 4, Manuela Truebano 4, Simon D. Rundle 4, Juraj Paule 5, Ingo Ebersberger 3,2, Markus Pfenninger 1,2 1 Molecular Ecology Group, Institute for Ecology, Evolution and Diversity, Goethe-University, Frankfurt am Main, Germany 2 Adaptation and Climate, Senckenberg Biodiversity and Climate Research Centre, Frankfurt am Main, Germany 3 Department for Applied Bioinformatics, Institute for Cell Biology and Neuroscience, Goethe- University, Frankfurt am Main, Germany 4 Marine Biology and Ecology Research Centre, Marine Institute, School of Marine Science and Engineering, Plymouth University, Plymouth, United Kingdom 5 Department of Botany and Molecular Evolution, Senckenberg Research Institute, Frankfurt am Main, Germany * Author for Correspondence: Senckenberg Biodiversity and Climate Research Centre, Senckenberganlage 25, 60325 Frankfurt am Main, Germany. Tel.: +49 (0)69 75 42 18 30, E-mail: [email protected] Data deposition: BioProject: PRJNA350764, SRA: SRP092167 1 bioRxiv preprint doi: https://doi.org/10.1101/087254; this version posted November 11, 2016. The copyright holder for this preprint (which was not certified by peer review) is the author/funder, who has granted bioRxiv a license to display the preprint in perpetuity. -
Habitat Preference and Population Ecology of Limpets Cellana
Hindawi Publishing Corporation Journal of Ecosystems Volume 2014, Article ID 874013, 6 pages http://dx.doi.org/10.1155/2014/874013 Research Article Habitat Preference and Population Ecology of Limpets Cellana karachiensis (Winckworth) and Siphonaria siphonaria (Sowerby) at Veraval Coast of Kathiawar Peninsula, India Julee Faladu, Bhavik Vakani, Paresh Poriya, and Rahul Kundu Department of Biosciences, Saurashtra University, Rajkot, Gujarat 360005, India Correspondence should be addressed to Rahul Kundu; [email protected] Received 7 May 2014; Revised 6 July 2014; Accepted 20 July 2014; Published 17 August 2014 Academic Editor: Wen-Cheng Liu Copyright © 2014 Julee Faladu et al. This is an open access article distributed under the Creative Commons Attribution License, which permits unrestricted use, distribution, and reproduction in any medium, provided the original work is properly cited. Present study reports the habitat preference and spatiotemporal variations in the population abundance of limpets Cellana karachiensis and Siphonaria siphonaria inhabiting rocky intertidal zones of Veraval coast, Kathiawar Peninsula, India. The entire intertidal zone of the Veraval coast was divided into five microsampling sites based on their substratum type and assemblage structure. Extensive field surveys were conducted every month in these microsampling sites and the population abundance of two limpet species was analyzed using belt transect method. The results revealed that C. karachiensis was the dominating species at microsampling Site-1 (having rocky substratum) possibly due to its ability to tolerate high desiccation, salinity, and temperature fluctuations, while the S. siphonaria was found to be the most dominating species at microsampling Site-2 (having rocky substratum with abundant algal population) possibly due to their preference for the perpetual wet areas. -
Rocky Shore Snails As Material for Projects (With a Key for Their Identification)
Field Studies, 10, (2003) 601 - 634 ROCKY SHORE SNAILS AS MATERIAL FOR PROJECTS (WITH A KEY FOR THEIR IDENTIFICATION) J. H. CROTHERS Egypt Cottage, Fair Cross, Washford, Watchet, Somerset TA23 0LY ABSTRACT Rocky sea shores are amongst the best habitats in which to carry out biological field projects. In that habitat, marine snails (prosobranchs) offer the most opportunities for individual investigations, being easy to find, to identify, to count and to measure and beng sufficiently robust to survive the experience. A key is provided for the identification of the larger species and suggestions are made for investigations to exploit selected features of individual species. INTRODUCTION Rocky sea shores offer one of the best habitats for individual or group investigations. Not only is there de facto public access (once you have got there) but also the physical factors that dominate the environment - tides (inundation versus desiccation), waves, heat, cold, light, dark, salinity etc. - change significantly over a few metres in distance. As a bonus, most of the fauna and flora lives out on the open rock surface and patterns of distribution may be clearly visible to the naked eye. Finally, they are amongst the most ‘natural’ of habitats in the British Isles; unless there has been an oil spill, rocky sea shores are unlikely to have been greatly affected by covert human activity. Some 270 species of marine snail (Phylum Mollusca, Class Gastropoda; Sub-Class Prosobranchia) live in the seas around the British Isles (Graham, 1988) and their empty shells may be found on many beaches. Most of these species are small (less than 3 mm long) or live beneath the tidemarks. -
MOLLUSCS Species Names – for Consultation 1
MOLLUSCS species names – for consultation English name ‘Standard’ Gaelic name Gen Scientific name Notes Neologisms in italics der MOLLUSC moileasg m MOLLUSCS moileasgan SEASHELL slige mhara f SEASHELLS sligean mara SHELLFISH (singular) maorach m SHELLFISH (plural) maoraich UNIVALVE SHELLFISH aon-mhogalach m (singular) UNIVALVE SHELLFISH aon-mhogalaich (plural) BIVALVE SHELLFISH dà-mhogalach m (singular) BIVALVE SHELLFISH dà-mhogalaich (plural) LIMPET (general) bàirneach f LIMPETS bàirnich common limpet bàirneach chumanta f Patella vulgata ‘common limpet’ slit limpet bàirneach eagach f Emarginula fissura ‘notched limpet’ keyhole limpet bàirneach thollta f Diodora graeca ‘holed limpet’ china limpet bàirneach dhromanach f Patella ulyssiponensis ‘ridged limpet’ blue-rayed limpet copan Moire m Patella pellucida ‘The Virgin Mary’s cup’ tortoiseshell limpet bàirneach riabhach f Testudinalia ‘brindled limpet’ testudinalis white tortoiseshell bàirneach bhàn f Tectura virginea ‘fair limpet’ limpet TOP SHELL brùiteag f TOP SHELLS brùiteagan f painted top brùiteag dhotamain f Calliostoma ‘spinning top shell’ zizyphinum turban top brùiteag thurbain f Gibbula magus ‘turban top shell’ grey top brùiteag liath f Gibbula cineraria ‘grey top shell’ flat top brùiteag thollta f Gibbula umbilicalis ‘holed top shell’ pheasant shell slige easaig f Tricolia pullus ‘pheasant shell’ WINKLE (general) faochag f WINKLES faochagan f banded chink shell faochag chlaiseach bhannach f Lacuna vincta ‘banded grooved winkle’ common winkle faochag chumanta f Littorina littorea ‘common winkle’ rough winkle (group) faochag gharbh f Littorina spp. ‘rough winkle’ small winkle faochag bheag f Melarhaphe neritoides ‘small winkle’ flat winkle (2 species) faochag rèidh f Littorina mariae & L. ‘flat winkle’ 1 MOLLUSCS species names – for consultation littoralis mudsnail (group) seilcheag làthaich f Fam. -
Population Characteristics of the Limpet Patella Caerulea (Linnaeus, 1758) in Eastern Mediterranean (Central Greece)
water Article Population Characteristics of the Limpet Patella caerulea (Linnaeus, 1758) in Eastern Mediterranean (Central Greece) Dimitris Vafidis, Irini Drosou, Kostantina Dimitriou and Dimitris Klaoudatos * Department of Ichthyology and Aquatic Environment, School of Agriculture Sciences, University of Thessaly, 38446 Volos, Greece; dvafi[email protected] (D.V.); [email protected] (I.D.); [email protected] (K.D.) * Correspondence: [email protected] Received: 27 February 2020; Accepted: 19 April 2020; Published: 21 April 2020 Abstract: Limpets are pivotal for structuring and regulating the ecological balance of littoral communities and are widely collected for human consumption and as fishing bait. Limpets of the species Patella caerulea were collected between April 2016 and April 2017 from two sites, and two samplings per each site with varying degree of exposure to wave action and anthropogenic pressure, in Eastern Mediterranean (Pagasitikos Gulf, Central Greece). This study addresses a knowledge gap on population characteristics of P. caerulea populations in Eastern Mediterranean, assesses population structure, allometric relationships, and reproductive status. Morphometric characteristics exhibited spatio-temporal variation. Population density was significantly higher at the exposed site. Spatial relationship between members of the population exhibited clumped pattern of dispersion during spring. Broadcast spawning of the population occurred during summer. Seven dominant age groups were identified, with the dominant cohort in the third-year -
Os Nomes Galegos Dos Moluscos 2020 2ª Ed
Os nomes galegos dos moluscos 2020 2ª ed. Citación recomendada / Recommended citation: A Chave (20202): Os nomes galegos dos moluscos. Xinzo de Limia (Ourense): A Chave. https://www.achave.ga /wp!content/up oads/achave_osnomesga egosdos"mo uscos"2020.pd# Fotografía: caramuxos riscados (Phorcus lineatus ). Autor: David Vilasís. $sta o%ra est& su'eita a unha licenza Creative Commons de uso a%erto( con reco)ecemento da autor*a e sen o%ra derivada nin usos comerciais. +esumo da licenza: https://creativecommons.org/ icences/%,!nc-nd/-.0/deed.g . Licenza comp eta: https://creativecommons.org/ icences/%,!nc-nd/-.0/ ega code. anguages. 1 Notas introdutorias O que cont!n este documento Neste recurso léxico fornécense denominacións para as especies de moluscos galegos (e) ou europeos, e tamén para algunhas das especies exóticas máis coñecidas (xeralmente no ámbito divulgativo, por causa do seu interese científico ou económico, ou por seren moi comúns noutras áreas xeográficas) ! primeira edición d" Os nomes galegos dos moluscos é do ano #$%& Na segunda edición (2$#$), adicionáronse algunhas especies, asignáronse con maior precisión algunhas das denominacións vernáculas galegas, corrixiuse algunha gralla, rema'uetouse o documento e incorporouse o logo da (have. )n total, achéganse nomes galegos para *$+ especies de moluscos A estrutura )n primeiro lugar preséntase unha clasificación taxonómica 'ue considera as clases, ordes, superfamilias e familias de moluscos !'uí apúntanse, de maneira xeral, os nomes dos moluscos 'ue hai en cada familia ! seguir -
Cuttlefish Sepia Officinalis (Mollusca-Cephalopoda)
Journal of Marine Science and Technology, Vol. 22, No. 1, pp. 15-24 (2014) 15 DOI: 10.6119/JMST-013-0820-1 NEUROGENESIS IN CEPHALOPODS: “ECO-EVO-DEVO” APPROACH IN THE CUTTLEFISH SEPIA OFFICINALIS (MOLLUSCA-CEPHALOPODA) Sandra Navet1, Sébastien Baratte2, 3, Yann Bassaglia2, 4, Aude Andouche2, Auxane Buresi2, and Laure Bonnaud1, 2 Key words: nervous system, cephalopods, development, evolution. from developmental processes and have been selected during evolution as they confer an adaptive advantage. In the general concern of Evo-Devo investigations, the ecological dimension ABSTRACT of the development is essential in the light of new knowledge Cephalopods are new evolutionary and ecological models. on genome plasticity [54]. Actually, since development is also By their phylogenetic position (Lophotrochozoa, Mollusca), influenced by non-genetic parameters, as environmental varia- they provide a missing master piece in the whole puzzle of tions or epigenetic processes, the functional and adaptive neurodevelopment studies. Their derived and specific nervous context of organisms to their environment has to be integrated system but also their convergence with vertebrates offer into a new field to study evolution of metazoans, called Eco- abundant materials to question the evolution and development Evo-Devo. of the nervous system of Metazoa (evo-devo studies). In Nervous system (NS) organisation of numerous metazoans addition, their various adaptions to different modes of life is well known but efforts on development are restricted to open new fields of investigation of developmental plasticity chordates and ecdysozoans: the mouse, Drosophila, Caenor- according to ecological context (eco-evo-devo approach). In habditis being the most extensively explored evo-devo models this paper, we review the recent works on cephalopod nervous [2]. -
Cephalopoda: Idiosepiidae) in Indonesian Waters
ZOBODAT - www.zobodat.at Zoologisch-Botanische Datenbank/Zoological-Botanical Database Digitale Literatur/Digital Literature Zeitschrift/Journal: Annalen des Naturhistorischen Museums in Wien Jahr/Year: 2007 Band/Volume: 108B Autor(en)/Author(s): Klepal Waltraud, von Byern J. Artikel/Article: Occurrence of Idiosepius pygmaeus (Cephalopoda, Idiosepiidae) in Indonesian waters. 137-144 ©Naturhistorisches Museum Wien, download unter www.biologiezentrum.at Ann. Naturhist. Mus. Wien 108 B 137- 144 Wien, Mai 2007 Occurrence of Idiosepius pygmaeus (Cephalopoda: Idiosepiidae) in Indonesian waters J. von Byern* & W. Klepal* Abstract Individuals of/, pygmaeus STEENSTRUP, 1881 have been rediscovered after more than 70 years at the type locality of/, pygmaeus hebereri GRIMPE, 1931 in Lombok, Indonesia. Occurrence of the animals between a flotsam of garbage indicates the ability to adapt to new habitats. Key words: Cephalopoda, Distribution, Idiosepiidae, Idiosepius pygmaeus, Indonesia Introduction Idiosepiidae are represented by a single genus with seven currently valid species, Idio- sepius biserialis Voss, 1962, /. macrocheir Voss, 1962, /. notoides BERRY, 1921, /. paradoxus (ORTMANN, 1888), /. picteti (JOUBIN, 1894), /. pygmaeus STEENSTRUP, 1881, /. thailandicus CHOTIYAPUTTA, OKUTANI & CHAITIAMVONG, 1991 (for a review see VON BOLETZKY & al. 2005). Their distribution stretches from Russia, Japan, the Indo- Pacific region to Tasmania as well as Moçambique (APPELLÖF 1898; SASAKI 1914; YAMAMOTO 1942; Voss 1962; OKUTANI 1973; Li 1983; Lu & PHILLIPS 1985; NATEE- WATHANA 1997; NESIS & al. 2002; VON BYERN & al. 2005). One conspicuous morpho- logical character of this genus is the adhesive organ located on the posterior part of the dorsal mantle side. This is used for attachment during the day to the lower leaf surfaces of sea grass or algae for camouflage.