Structural Insights Into Ring Formation of Cohesin and Related Smc Complexes Thomas Gligoris1,* and Jan Löwe2
Total Page:16
File Type:pdf, Size:1020Kb
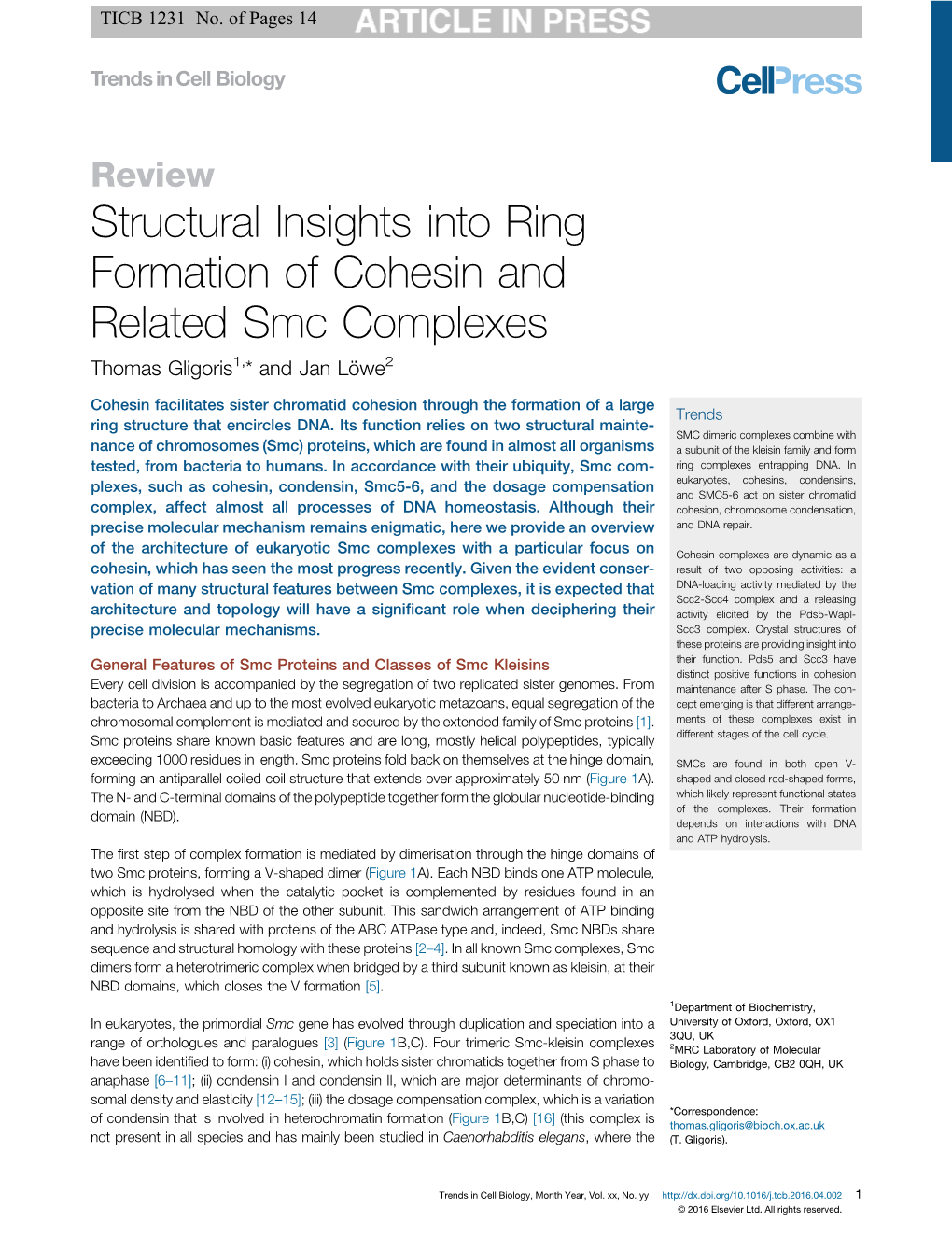
Load more
Recommended publications
-
Global Characteristics of Csig-Associated Gene Expression Changes in Human Hek293 Cells and the Implications for Csig Regulating Cell Proliferation and Senescence
View metadata, citation and similar papers at core.ac.uk brought to you by CORE provided by Frontiers - Publisher Connector ORIGINAL RESEARCH published: 15 May 2015 doi: 10.3389/fendo.2015.00069 Global characteristics of CSIG-associated gene expression changes in human HEK293 cells and the implications for CSIG regulating cell proliferation and senescence Liwei Ma, Wenting Zhao, Feng Zhu, Fuwen Yuan, Nan Xie, Tingting Li, Pingzhang Wang and Tanjun Tong* Research Center on Aging. Department of Biochemistry and Molecular Biology, School of Basic Medical Sciences, Peking University Health Science Center, Beijing Key Laboratory of Protein Posttranslational Modifications and Cell Function, Edited by: Beijing, China Wen Zhou, Columbia University, USA Reviewed by: Cellular senescence-inhibited gene (CSIG), also named as ribosomal_L1 domain-contain- Jian Zhong, ing 1 (RSL1D1), is implicated in various processes including cell cycle regulation, cellular Mayo Clinic, USA Xiaoxu Zheng, senescence, apoptosis, and tumor metastasis. However, little is known about the regulatory University of Maryland Baltimore, USA mechanism underlying its functions. To screen important targets and signaling pathways Wensi Tao, University of Miami, USA modulated by CSIG, we compared the gene expression profiles in CSIG-silencing and control *Correspondence: HEK293 cells using Affymetrix microarray Human Genome U133 Plus 2.0 GeneChips. A Tanjun Tong, total of 590 genes displayed statistically significant expression changes, with 279 genes Department of Biochemistry and up-regulated and 311 down-regulated, respectively. These genes are involved in a broad array Molecular Biology, Research Center on Aging, Peking University Health of biological processes, mainly in transcriptional regulation, cell cycle, signal transduction, Science Center, 38 Xueyuan Road, oxidation reduction, development, and cell adhesion. -
Supplementary Table S1. Upregulated Genes Differentially
Supplementary Table S1. Upregulated genes differentially expressed in athletes (p < 0.05 and 1.3-fold change) Gene Symbol p Value Fold Change 221051_s_at NMRK2 0.01 2.38 236518_at CCDC183 0.00 2.05 218804_at ANO1 0.00 2.05 234675_x_at 0.01 2.02 207076_s_at ASS1 0.00 1.85 209135_at ASPH 0.02 1.81 228434_at BTNL9 0.03 1.81 229985_at BTNL9 0.01 1.79 215795_at MYH7B 0.01 1.78 217979_at TSPAN13 0.01 1.77 230992_at BTNL9 0.01 1.75 226884_at LRRN1 0.03 1.74 220039_s_at CDKAL1 0.01 1.73 236520_at 0.02 1.72 219895_at TMEM255A 0.04 1.72 201030_x_at LDHB 0.00 1.69 233824_at 0.00 1.69 232257_s_at 0.05 1.67 236359_at SCN4B 0.04 1.64 242868_at 0.00 1.63 1557286_at 0.01 1.63 202780_at OXCT1 0.01 1.63 1556542_a_at 0.04 1.63 209992_at PFKFB2 0.04 1.63 205247_at NOTCH4 0.01 1.62 1554182_at TRIM73///TRIM74 0.00 1.61 232892_at MIR1-1HG 0.02 1.61 204726_at CDH13 0.01 1.6 1561167_at 0.01 1.6 1565821_at 0.01 1.6 210169_at SEC14L5 0.01 1.6 236963_at 0.02 1.6 1552880_at SEC16B 0.02 1.6 235228_at CCDC85A 0.02 1.6 1568623_a_at SLC35E4 0.00 1.59 204844_at ENPEP 0.00 1.59 1552256_a_at SCARB1 0.02 1.59 1557283_a_at ZNF519 0.02 1.59 1557293_at LINC00969 0.03 1.59 231644_at 0.01 1.58 228115_at GAREM1 0.01 1.58 223687_s_at LY6K 0.02 1.58 231779_at IRAK2 0.03 1.58 243332_at LOC105379610 0.04 1.58 232118_at 0.01 1.57 203423_at RBP1 0.02 1.57 AMY1A///AMY1B///AMY1C///AMY2A///AMY2B// 208498_s_at 0.03 1.57 /AMYP1 237154_at LOC101930114 0.00 1.56 1559691_at 0.01 1.56 243481_at RHOJ 0.03 1.56 238834_at MYLK3 0.01 1.55 213438_at NFASC 0.02 1.55 242290_at TACC1 0.04 1.55 ANKRD20A1///ANKRD20A12P///ANKRD20A2/// -
S41467-020-18249-3.Pdf
ARTICLE https://doi.org/10.1038/s41467-020-18249-3 OPEN Pharmacologically reversible zonation-dependent endothelial cell transcriptomic changes with neurodegenerative disease associations in the aged brain Lei Zhao1,2,17, Zhongqi Li 1,2,17, Joaquim S. L. Vong2,3,17, Xinyi Chen1,2, Hei-Ming Lai1,2,4,5,6, Leo Y. C. Yan1,2, Junzhe Huang1,2, Samuel K. H. Sy1,2,7, Xiaoyu Tian 8, Yu Huang 8, Ho Yin Edwin Chan5,9, Hon-Cheong So6,8, ✉ ✉ Wai-Lung Ng 10, Yamei Tang11, Wei-Jye Lin12,13, Vincent C. T. Mok1,5,6,14,15 &HoKo 1,2,4,5,6,8,14,16 1234567890():,; The molecular signatures of cells in the brain have been revealed in unprecedented detail, yet the ageing-associated genome-wide expression changes that may contribute to neurovas- cular dysfunction in neurodegenerative diseases remain elusive. Here, we report zonation- dependent transcriptomic changes in aged mouse brain endothelial cells (ECs), which pro- minently implicate altered immune/cytokine signaling in ECs of all vascular segments, and functional changes impacting the blood–brain barrier (BBB) and glucose/energy metabolism especially in capillary ECs (capECs). An overrepresentation of Alzheimer disease (AD) GWAS genes is evident among the human orthologs of the differentially expressed genes of aged capECs, while comparative analysis revealed a subset of concordantly downregulated, functionally important genes in human AD brains. Treatment with exenatide, a glucagon-like peptide-1 receptor agonist, strongly reverses aged mouse brain EC transcriptomic changes and BBB leakage, with associated attenuation of microglial priming. We thus revealed tran- scriptomic alterations underlying brain EC ageing that are complex yet pharmacologically reversible. -
The Emerging Role of Cohesin in the DNA Damage Response
G C A T T A C G G C A T genes Review The Emerging Role of Cohesin in the DNA Damage Response Ireneusz Litwin * , Ewa Pilarczyk and Robert Wysocki Institute of Experimental Biology, University of Wroclaw, 50-328 Wroclaw, Poland; [email protected] (E.P.); [email protected] (R.W.) * Correspondence: [email protected]; Tel.: +48-71-375-4126 Received: 29 October 2018; Accepted: 21 November 2018; Published: 28 November 2018 Abstract: Faithful transmission of genetic material is crucial for all organisms since changes in genetic information may result in genomic instability that causes developmental disorders and cancers. Thus, understanding the mechanisms that preserve genome integrity is of fundamental importance. Cohesin is a multiprotein complex whose canonical function is to hold sister chromatids together from S-phase until the onset of anaphase to ensure the equal division of chromosomes. However, recent research points to a crucial function of cohesin in the DNA damage response (DDR). In this review, we summarize recent advances in the understanding of cohesin function in DNA damage signaling and repair. First, we focus on cohesin architecture and molecular mechanisms that govern sister chromatid cohesion. Next, we briefly characterize the main DDR pathways. Finally, we describe mechanisms that determine cohesin accumulation at DNA damage sites and discuss possible roles of cohesin in DDR. Keywords: cohesin; cohesin loader; DNA double-strand breaks; replication stress; DNA damage tolerance 1. Introduction Genomes of all living organisms are continuously challenged by endogenous and exogenous insults that threaten genome stability. It has been estimated that human cells suffer more than 70,000 DNA lesions per day, most of which are single-strand DNA breaks (SSBs) [1]. -
RESEARCH ARTICLE Regulatory Network of Micrornas, Target
DOI:http://dx.doi.org/10.7314/APJCP.2015.16.2.475 Regulatory Network of MicroRNAs, Target Genes, Transcription Factors and Host Genes in Endometrial Cancer RESEARCH ARTICLE Regulatory Network of MicroRNAs, Target Genes, Transcription Factors and Host Genes in Endometrial Cancer Lu-Chen Xue1,3, Zhi-Wen Xu2,3*, Kun-Hao Wang2,3, Ning Wang2,3, Xiao-Xu Zhang1, Shang Wang2,3 Abstract Genes and microRNAs (miRNAs) have important roles in human oncology. However, most of the biological factors are reported in disperse form which makes it hard to discover the pathology. In this study, genes and miRNAs involved in human endometrial cancer(EC) were collected and formed into regulatory networks following their interactive relations, including miRNAs targeting genes, transcription factors (TFs) regulating miRNAs and miRNAs included in their host genes. Networks are constructed hierarchically at three levels: differentially expressed, related and global. Among the three, the differentially expressed network is the most important and fundamental network that contains the key genes and miRNAs in EC. The target genes, TFs and miRNAs are differentially expressed in EC so that any mutation in them may impact on EC development. Some key pathways in networks were highlighted to analyze how they interactively influence other factors and carcinogenesis. Upstream and downstream pathways of the differentially expressed genes and miRNAs were compared and analyzed. The purpose of this study was to partially reveal the deep regulatory mechanisms in EC using a new method that combines comprehensive genes and miRNAs together with their relationships. It may contribute to cancer prevention and gene therapy of EC. -
Original Article Rnai Screening Identifies KAT8 As a Key Molecule Important for Cancer Cell Survival
Int J Clin Exp Pathol 2013;6(5):870-877 www.ijcep.com /ISSN:1936-2625/IJCEP1302018 Original Article RNAi screening identifies KAT8 as a key molecule important for cancer cell survival Shuang Zhang1,2, Xianhong Liu2, Yong Zhang1, Ying Cheng2*, Yang Li1* 1Department of Pathophysiology, Norman Bathune College of Medical Sciences, Jilin University, Changchun, China; 2The first Department of Internal Medicine, Jilin Province Cancer Hospital, Changchun, China. *Equal contri- bution. Received February 16, 2013; Accepted April 1, 2013; Epub April 15, 2013; Published April 30, 2013 Abstract: Histone acetyltransferases (HATs) regulate many critical cancer events, including transcriptional regula- tion of oncogene and tumor suppressors, chromatin structure and DNA damage response. Abnormal expression of HATs has been reported in a number of cancers. However, cellular functions of HATs in cancer and molecular mechanisms remain largely unclear. Here, we performed a lentiviral vector-mediated RNAi screen to systematically address the function of HATs in lung cancer cell growth and viability. We identified 8 HATs genes involved in A549 cell viability. Further experiments showed that KAT8 regulates G2/M cell cycle arrest through AKT/ERK-cyclin D1 signaling. Moreover, KAT8 inhibition led to p53 induction and subsequently reduced bcl-2 expression. Our results demonstrate an important role of KAT8 in cancer and suggest that KAT8 could be a novel cancer therapeutic target. Keywords: HATs, RNAi screen, KAT8, cyclin D1, p53, cell survival Introduction well as DNA replication. Moreover, HATs gene expression profiles have been reported to be Histone acetylation, one of the most extensive- associated with pathological and clinical out- ly characterized epigenetic modifications, is comes in breast cancer [6]. -
Microorganisms
microorganisms Review Rules and Exceptions: The Role of Chromosomal ParB in DNA Segregation and Other Cellular Processes Adam Kawalek y , Pawel Wawrzyniak y, Aneta Agnieszka Bartosik and Grazyna Jagura-Burdzy * Department of Microbial Biochemistry, Institute of Biochemistry and Biophysics, Polish Academy of Sciences, Pawi´nskiego5a, 02-106 Warsaw, Poland; [email protected] (A.K.); [email protected] (P.W.); [email protected] (A.A.B.) * Correspondence: [email protected]; Tel.: +48-225921212 These authors contributed equally to this work. y Received: 4 December 2019; Accepted: 9 January 2020; Published: 11 January 2020 Abstract: The segregation of newly replicated chromosomes in bacterial cells is a highly coordinated spatiotemporal process. In the majority of bacterial species, a tripartite ParAB-parS system, composed of an ATPase (ParA), a DNA-binding protein (ParB), and its target(s) parS sequence(s), facilitates the initial steps of chromosome partitioning. ParB nucleates around parS(s) located in the vicinity of newly replicated oriCs to form large nucleoprotein complexes, which are subsequently relocated by ParA to distal cellular compartments. In this review, we describe the role of ParB in various processes within bacterial cells, pointing out interspecies differences. We outline recent progress in understanding the ParB nucleoprotein complex formation and its role in DNA segregation, including ori positioning and anchoring, DNA condensation, and loading of the structural maintenance of chromosome (SMC) proteins. The auxiliary roles of ParBs in the control of chromosome replication initiation and cell division, as well as the regulation of gene expression, are discussed. Moreover, we catalog ParB interacting proteins. -
Comprehensive Biological Information Analysis of PTEN Gene in Pan-Cancer
Comprehensive biological information analysis of PTEN gene in pan-cancer Hang Zhang Shanghai Medical University: Fudan University https://orcid.org/0000-0002-5853-7754 Wenhan Zhou Shanghai Medical University: Fudan University Xiaoyi Yang Shanghai Jiao Tong University School of Medicine Shuzhan Wen Shanghai Medical University: Fudan University Baicheng Zhao Shanghai Medical University: Fudan University Jiale Feng Shanghai Medical University: Fudan University Shuying Chen ( [email protected] ) https://orcid.org/0000-0002-9215-9777 Primary research Keywords: PTEN, correlated genes, TCGA, GEPIA, UALCAN, GTEx, expression, cancer Posted Date: April 12th, 2021 DOI: https://doi.org/10.21203/rs.3.rs-388887/v1 License: This work is licensed under a Creative Commons Attribution 4.0 International License. Read Full License Page 1/21 Abstract Background PTEN is a multifunctional tumor suppressor gene mutating at high frequency in a variety of cancers. However, its expression in pan-cancer, correlated genes, survival prognosis, and regulatory pathways are not completely described. Here, we aimed to conduct a comprehensive analysis from the above perspectives in order to provide reference for clinical application. Methods we studied the expression levels in cancers by using data from TCGA and GTEx database. Obtain expression box plot from UALCAN database. Perform mutation analysis on the cBioportal website. Obtain correlation genes on the GEPIA website. Construct protein network and perform KEGG and GO enrichment analysis on the STRING database. Perform prognostic analysis on the Kaplan-Meier Plotter website. We also performed transcription factor prediction on the PROMO database and performed RNA-RNA association and RNA-protein interaction on the RNAup Web server and RPISEq. -
Análisis Funcional De MCPH1 En La Condensación Cromosómica Y El Control Del Ciclo Celular
Área de Genética, Departamento de Biología Experimental, Facultad de Ciencias Experimentales, Universidad de Jaén. Análisis funcional de MCPH1 en la condensación cromosómica y el control del ciclo celular TESIS DOCTORAL María de la Cabeza Arroyo López Jaén 2018 Área de Genética Departamento de Biología Experimental Facultad de Ciencias Experimentales Universidad de Jaén TESIS DOCTORAL Análisis funcional de MCPH1 en la condensación cromosómica y el control del ciclo celular MARIA DE LA CABEZA ARROYO LOPEZ 2018 Análisis funcional de MCPH1 en la condensación cromosómica y el control del ciclo celular Dirigida por los doctores: J. Alberto Marchal Ortega Antonio Sánchez Baca Memoria presentada por la licenciada María de la Cabeza Arroyo López para optar al Grado de Doctor Internacional en Biología. Junio, 2018 El presente trabajo ha sido realizado en el Área de Genética del Departamento de Biología Experimental de la Universidad de Jaén durante los años 2014-2018, período durante el cual el Doctorando disfrutó de una Ayuda para la Formación de Personal Investigador con cargo al Plan de Apoyo a la Investigación, Desarrollo Tecnológico e Innovación de la Universidad de Jaén. Además, parte de este trabajo se ha llevado a cabo en el “Department of Genetics, Cell Biology and Developoment, University of Minnesota”, gracias a unas ayudas para estancias breves proporcionadas por la organización EMBO y la Escuela de Doctorado de la Universidad de Jaén. La investigación realizada ha sido financiada en parte por la Universidad de Jaén a través del proyecto UJA/2011/12/36, y la Junta de Andalucía a través del programa “Ayudas a grupos de Investigación (BIO-220). -
Esco1 and Esco2 Regulate Distinct Cohesin Functions During Cell Cycle Progression
Esco1 and Esco2 regulate distinct cohesin functions during cell cycle progression Reem M. Alomera,1, Eulália M. L. da Silvab,1, Jingrong Chenb, Katarzyna M. Piekarzb, Katherine McDonaldb, Courtney G. Sansamb, Christopher L. Sansama,b, and Susannah Rankina,b,2 aDepartment of Cell Biology, University of Oklahoma Health Sciences Center, Oklahoma City, OK 73104; and bProgram in Cell Cycle and Cancer Biology, Oklahoma Medical Research Foundation, Oklahoma City, OK 73104 Edited by Douglas Koshland, University of California, Berkeley, CA, and approved July 31, 2017 (received for review May 19, 2017) Sister chromatids are tethered together by the cohesin complex from Finally, chromatin immunoprecipitation experiments in somatic the time they are made until their separation at anaphase. The ability cells indicate that Esco1 and Esco2 have distinct chromosomal of cohesin to tether sister chromatids together depends on acetyla- addresses. Colocalization of Esco1 with the insulator protein tion of its Smc3 subunit by members of the Eco1 family of cohesin CTCF and cohesin at the base of chromosome loops suggests that acetyltransferases. Vertebrates express two orthologs of Eco1, called Esco1 promotes normal chromosome structure (14, 15). Consistent Esco1 and Esco2, both of which are capable of modifying Smc3, but with this, depletion of Esco1 in somatic cells results in dysregulated their relative contributions to sister chromatid cohesion are unknown. transcriptional profiles (15). In contrast, Esco2 is localized to dis- We therefore set out to determine the precise contributions of Esco1 tinctly different sites, perhaps due to association with the CoREST and Esco2 to cohesion in vertebrate cells. Here we show that cohesion repressive complex (15, 16). -
The Anti-Angiogenic Effects of Sparstolonin B Henry Rhodes Bateman University of South Carolina
University of South Carolina Scholar Commons Theses and Dissertations 1-1-2013 The Anti-Angiogenic Effects of Sparstolonin B Henry Rhodes Bateman University of South Carolina Follow this and additional works at: https://scholarcommons.sc.edu/etd Part of the Biomedical Commons, and the Chemicals and Drugs Commons Recommended Citation Bateman, H. R.(2013). The Anti-Angiogenic Effects of Sparstolonin B. (Doctoral dissertation). Retrieved from https://scholarcommons.sc.edu/etd/2090 This Open Access Dissertation is brought to you by Scholar Commons. It has been accepted for inclusion in Theses and Dissertations by an authorized administrator of Scholar Commons. For more information, please contact [email protected]. THE ANTI -ANGIOGENIC EFFECTS OF SPARSTOLONIN B by Henry Rhodes Bateman, III Bachelor of Science Virginia Polytechnic Institute and State University, 2000 Master of Science Virginia Commonwealth University, 2003 Submitted in Partial Fulfillment of the Requirements For the Degree of Doctor of Philosophy in Biomedical Science School of Medicine University of South Carolina 2013 Accepted by: Susan M. Lessner, Major Professor Daping Fan, Chairman, Examining Committee Francis G. Spinale, Committee Member Ugra S. Singh, Committee Member Rekha C. Patel, Committee Member Lacy Ford, Vice Provost and Dean of Graduate Studies © Copyright by Henry Bateman, 2013 All Rights Reserved. ii DEDICATION I would like to dedicate this work to my family. My family has been extremely supportive and helpful during my completion of this program. My parents have always been devoted to my siblings and me, sacrificing everything to help us realize our dreams. My mother, who passed away seven years ago, was the greatest teacher in my life. -
Structural Analysis of the Cohesin Complex Yan Li
Structural analysis of the cohesin complex Yan Li To cite this version: Yan Li. Structural analysis of the cohesin complex. Structural Biology [q-bio.BM]. Université Grenoble Alpes, 2019. English. NNT : 2019GREAV012. tel-03187406 HAL Id: tel-03187406 https://tel.archives-ouvertes.fr/tel-03187406 Submitted on 1 Apr 2021 HAL is a multi-disciplinary open access L’archive ouverte pluridisciplinaire HAL, est archive for the deposit and dissemination of sci- destinée au dépôt et à la diffusion de documents entific research documents, whether they are pub- scientifiques de niveau recherche, publiés ou non, lished or not. The documents may come from émanant des établissements d’enseignement et de teaching and research institutions in France or recherche français ou étrangers, des laboratoires abroad, or from public or private research centers. publics ou privés. THESIS / THÈSE To obtain the title of / Pour obtenir le grade de DOCTEUR DE L’UNIVERSITÉ GRENOBLE ALPES Discipline / Spécialité : Structure Biology and Nanotechnology Arrêté ministériel : 7 août 2006 Presented by / Présentée par Yan LI Thesis supervisor / Thèse dirigée par Dr. Daniel Panne Thesis prepared at / Thèse préparée au sein du European Molecular Biology Laboratory (EMBL), Grenoble/ In / Dans l'École Doctorale de Chimie et Science du Vivant Structural analysis of the Cohesin Complex / Analyse structurale du complexe de la cohésine Public defense on / Thèse soutenue publiquement le 01/04/2019 Jury members / devant le jury composé de : Dr. Benjamin ROWLAND Reviewer / Rapporteur Dr. Camilla BJORKEGREN Reviewer / Rapporteur Dr. Ramesh PILLAI President / Président Dr. Joanna TIMMINS Examiner / Examinateur 1 Table of Contents 2 Table of Contents ....................................................................................................................... 2 List of Abbreviations ................................................................................................................