In Vitro Environmental Toxicology - Concepts, Application and Assessment 157 Advances in Biochemical Engineering/Biotechnology
Total Page:16
File Type:pdf, Size:1020Kb
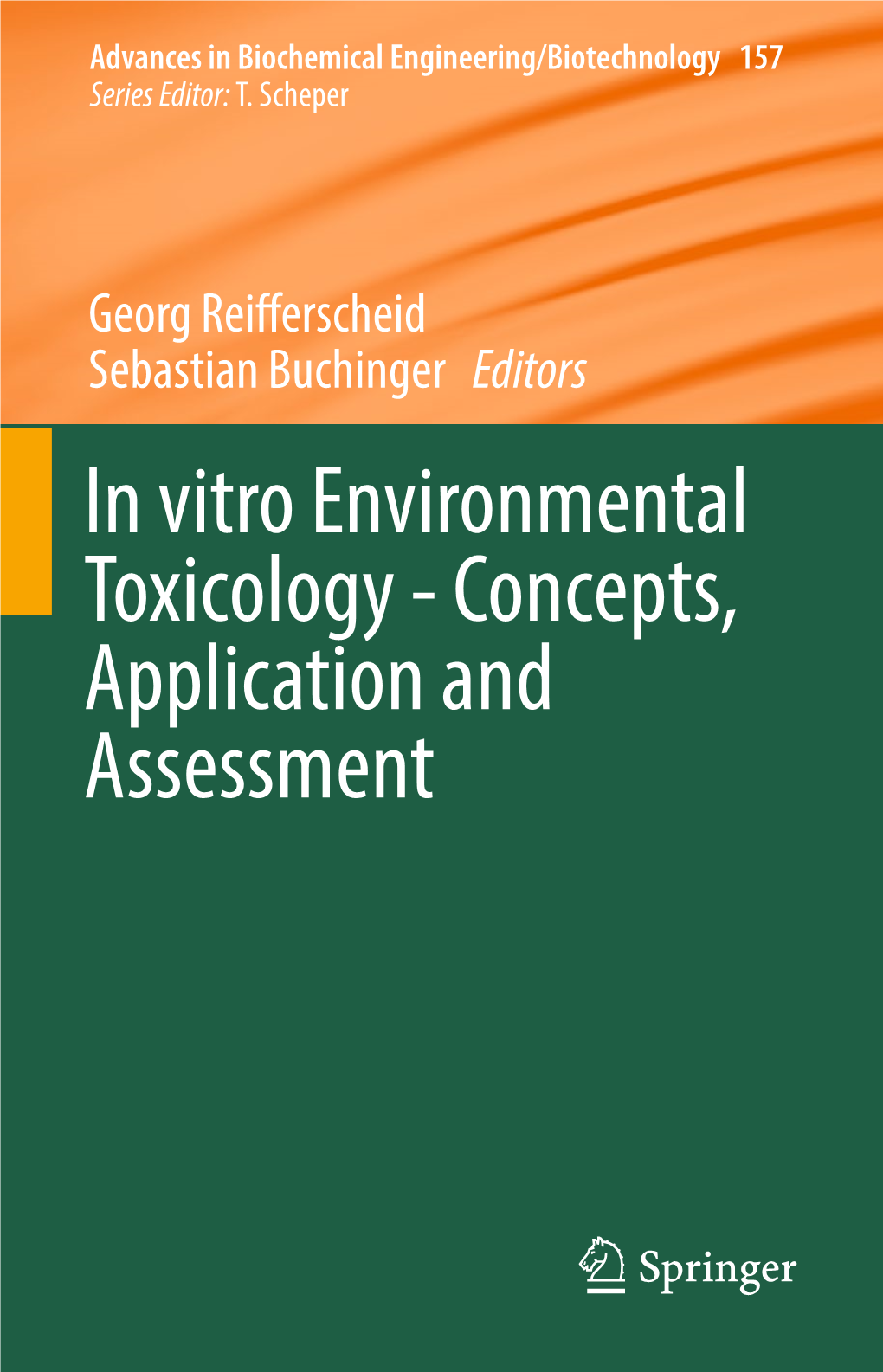
Load more
Recommended publications
-
Chapter 2 Studies Towards the Synthesis of Jlnhydride (Based Naturac (Products £ CQ 2A
Chapter 2 Studies towards the Synthesis of JLnhydride (Based NaturaC (Products _£ CQ 2A. Section A 9/LaCeic Anhydrides andJdomophthatic Anhydrides in Organic Synthesis \J This section features the following topics: 2A. 1 Maleic Anhydride and Derivatives: An Overview 2A.2 Homophthalic Anhydrides and their Applications 2 A3 References 2A.1 Section A: I. Maleic Anhydride and Derivatives: An Overview 2A.1.1 Introduction & Monoalkylmaleic Anhydrides Maleic anhydride (2,5-furandione) was prepared for the first time two centuries ago and became commercially available a century later by the catalytic oxidation of benzene using vanadium pentoxide.1 It is a versatile synthon wherein all the sites are amenable for a variety of reactions and possesses exceptional selectivity in reactions towards several nucleophiles. The vast array of nucleophilic reactions undergone by maleic anhydrides confer a high synthetic potential on them.2 In the past century, several symmetrically and unsymmetrically substituted maleic anhydride derivatives have been prepared, the simplest of them being methylmaleic anhydride or citraconic anhydride (1). Although the utilities of methylmaleic anhydride (1) have been well proven in laboratory as well as in industrial practice,3 only three synthetic approaches towards methylmaleic anhydride are known in the literature: (i) starting from citric acid by double dehydrative decarboxylation and isomerization,4 (ii) from ethyl acetoacetate via cyanohydrin formation followed by dehydrative cyclization5 and (iii) by the gas phase -
Methods of Isolation and Bioactivity of Alkaloids Obtained from Selected
DOI: 10.2478/cipms-2021-0016 Curr. Issues Pharm. Med. Sci., Vol. 34, No. 2, Pages 81-86 Current Issues in Pharmacy and Medical Sciences Formerly ANNALES UNIVERSITATIS MARIAE CURIE-SKLODOWSKA, SECTIO DDD, PHARMACIA journal homepage: http://www.curipms.umlub.pl/ Methods of isolation and bioactivity of alkaloids obtained from selected species belonging to the Amaryllidaceae and Lycopodiaceae families Aleksandra Dymek* , Tomasz Mroczek Independent Laboratory of Chemistry of Natural Products, The Chair of Pharmacognosy, Medical University of Lublin, Poland ARTICLE INFO ABSTRACT Received 17 February 2021 Alkaloids obtained from plants belonging to the Amaryllidaceae and Lycopodiaceae Accepted 20 May 2021 families are of great interest due to their numerous properties. They play a very important Keywords: role mainly due to their strong antioxidant, anxiolytic and anticholinesterase activities. Lycopodium sp., The bioactive compounds obtained from these two families, especially galanthamine Narcissus sp., and huperzine A, have found application in the treatment of the common and AChE inhibitors, TLC, incurable dementia-like Alzheimer’s disease. Thanks to this discovery, there has been SPE, a breakthrough in its treatment by significantly improving the patient’s quality of life and PLE, slowing down disease symptoms – albeit with no chance of a complete cure. Therefore, TLC-bioatography. a continuous search for new compounds with potent anti-AChE activity is needed in modern medicine. In obtaining new therapeutic bioactive phytochemicals from plant material, the isolation process and its efficiency are crucial. Many techniques are known for isolating bioactive compounds and determining their amounts in complex samples. The most commonly utilized methods are extraction using different variants of organic solvents allied with chromatographic and spectrometric techniques. -
200916697 Apr2017.Pdf
A metabolomics and transcriptomics comparison of Narcissus pseudonarcissus cv. Carlton field and in vitro tissues in relation to alkaloid production Aleya Ferdausi The University of Liverpool April 2017 Thesis submitted in accordance with the requirements of the University of Liverpool for the degree of Doctor in Philosophy by Aleya Ferdausi Acknowledgements First, I would like to express my profound gratitude to my supervisor Dr Meriel Jones for her continuous support, motivation, suggestions, and guidelines to continue my project work smoothly. I am also grateful to her for giving critical evaluation on my thesis. I would also like to thank my secondary supervisor Professor Anthony Hall for his support and guidelines to lead me on the bioinformatics discovery. I would like to thank my PhD assessors Professor Martin Mortimer and Dr James Hartwell for their valuable suggestions and feedback on my annual project progress. My sincere acknowledgements also goes to Dr Xianmin Chang for his entire help throughout the major part of my research such as providing Narcissus bulbs, tissue culture and alkaloid analysis method development, calculations and data interpretations. Mark Preston, Centre of Proteome analysis for helping with GC-MS analysis and Dr Phelan Marie, NMR Centre, University of Liverpool for helping with NMR analysis. Dr Ryan Joynson, for his helps regarding transcript annotation. Centre of Genomic Research, University of Liverpool, for RNA-sequencing. Dr Jane Pulman for her helps to learn basic molecular biology techniques. My sincere thanks are given to Jean Wood, Senior Technician, Lab G, Institute of Integrative Biology. I am also grateful to all other staff, research groups, post-graduate students and all members of the Biosciences building, University of Liverpool for their friendly and humble attitude, which made me feel my work place like home. -
Diversity of the Mountain Flora of Central Asia with Emphasis on Alkaloid-Producing Plants
diversity Review Diversity of the Mountain Flora of Central Asia with Emphasis on Alkaloid-Producing Plants Karimjan Tayjanov 1, Nilufar Z. Mamadalieva 1,* and Michael Wink 2 1 Institute of the Chemistry of Plant Substances, Academy of Sciences, Mirzo Ulugbek str. 77, 100170 Tashkent, Uzbekistan; [email protected] 2 Institute of Pharmacy and Molecular Biotechnology, Heidelberg University, Im Neuenheimer Feld 364, 69120 Heidelberg, Germany; [email protected] * Correspondence: [email protected]; Tel.: +9-987-126-25913 Academic Editor: Ipek Kurtboke Received: 22 November 2016; Accepted: 13 February 2017; Published: 17 February 2017 Abstract: The mountains of Central Asia with 70 large and small mountain ranges represent species-rich plant biodiversity hotspots. Major mountains include Saur, Tarbagatai, Dzungarian Alatau, Tien Shan, Pamir-Alai and Kopet Dag. Because a range of altitudinal belts exists, the region is characterized by high biological diversity at ecosystem, species and population levels. In addition, the contact between Asian and Mediterranean flora in Central Asia has created unique plant communities. More than 8100 plant species have been recorded for the territory of Central Asia; about 5000–6000 of them grow in the mountains. The aim of this review is to summarize all the available data from 1930 to date on alkaloid-containing plants of the Central Asian mountains. In Saur 301 of a total of 661 species, in Tarbagatai 487 out of 1195, in Dzungarian Alatau 699 out of 1080, in Tien Shan 1177 out of 3251, in Pamir-Alai 1165 out of 3422 and in Kopet Dag 438 out of 1942 species produce alkaloids. The review also tabulates the individual alkaloids which were detected in the plants from the Central Asian mountains. -
Chapter 2: Synthesis of Amaryllidaceae Alkaloids (Ismine, Trisphaeridine and Bicolorine)
CHAPTER 2: SYNTHESIS OF AMARYLLIDACEAE ALKALOIDS (ISMINE, TRISPHAERIDINE AND BICOLORINE) 126 2.1 Introduction Plants and natural products have played a long a crucial role in the treatment of various illnesses. They provide valuable sources of compounds with a wide variety of chemical structures and biological activities and have provided important prototypes for the development of novel drugs.1 It is impossible to overrate the importance of natural extracts as potential sources of new drugs. It is estimated that the plant kingdom comprises about 250,000 species, of which approximately 7% have been studied for biological activity and about 17% phytochemically.2 The Amaryllidaceae family consists of about 75 genera, whose 1100 species are widely spread in several countries around the world. Plants from the Amaryllidaceae family are used for the production of volatile oil. They are also cultivated as ornamental plants for their beautiful flowers. Amaryllidaceae plants are extensively used in traditional medicine throughout the tropics. They are used for their pharmacological effects and are frequently associated with several typical synthesized alkaloids.3 With the isolation of lycorine from N. pseudonarcissus,4 the study of Amaryllidaceae alkaloids began in 1877 and the interest around this group of naturally occurring compounds has increased with time because of their effective antitumoral and antiviral activities. Lycorine (3) (Figure 1) is a pyrrolo[de] phenathridine ring type alkaloid extracted from different Amaryllidaceae species, -
Université Du Québec
UNIVERSITÉ DU QUÉBEC THÈSE PRÉSENTÉE À L'UNIVERSITÉ DU QUÉBEC À TROIS-RIVIÈRES COMME EXIGENCE PARTIELLE DU DOCTORAT EN BIOLOGIE CELLULAIRE ET MOLÉCULAIRE PAR APARNA SINGH DISCOVERY OF NOVEL GENES AND ENZYMES INVOLVED IN AMARYLLIDACEAE ALKALOID BIOSYNTHESIS USING INTEGRATED MET ABOLOMICS AND TRANSCRIPTOMICS IN NARCISSUS PSEUDONARCISSUS 'KING ALFRED' JUIN 2018 Université du Québec à Trois-Rivières Service de la bibliothèque Avertissement L’auteur de ce mémoire ou de cette thèse a autorisé l’Université du Québec à Trois-Rivières à diffuser, à des fins non lucratives, une copie de son mémoire ou de sa thèse. Cette diffusion n’entraîne pas une renonciation de la part de l’auteur à ses droits de propriété intellectuelle, incluant le droit d’auteur, sur ce mémoire ou cette thèse. Notamment, la reproduction ou la publication de la totalité ou d’une partie importante de ce mémoire ou de cette thèse requiert son autorisation. UNIVERSITÉ DU QUÉBEC À TROIS-RIVIÈRES Cette thèse a été dirigée par: Isabel Desgagné-Penix, Ph. D. Université du Québec à Trois-Rivières Directrice de recherche Institution à laquelle se rattache l' évaluateur Jury d'évaluation de la thèse: Isabel Desgagné-Penix, Ph. D. Université du Québec à Trois-Rivières Prénom et nom, grade Institution à laquelle se rattache l' évaluateur Hugo Germain, Ph. D. Université du Québec à Trois-Rivières Prénom et nom, grade Institution à laquelle se rattache l' évaluateur Céline Van Themsche, Ph. D. Université du Québec à Trois-Rivières Prénom et nom, grade Institution à laquelle se rattache l' évaluateur Charles Goulet, Ph. D. Université Laval Prénom et nom, grade Institution à laquelle se rattache l' évaluateur Thèse soutenue le 28 mai 2018 To my parents and husband who were my strength in this journey IV ACKNOWLEDGEMENTS l am thankful to my research supervisor Isabel Desgagné-Penix for considering me in her laboratory and acquainted me enthusiastically to the field of plant specialized metabolism. -
An Overview on Potential Neuroprotective Compounds for Management of Alzheimer’S Disease
Send Orders of Reprints at [email protected] 1006 CNS & Neurological Disorders - Drug Targets, 2012, 11, 1006-1011 An Overview on Potential Neuroprotective Compounds for Management of Alzheimer’s Disease Ishfaq Ahmed Sheikh1,§, Riyasat Ali2,§, Tanveer A. Dar 3 and Mohammad Amjad Kamal*,1 1King Fahd Medical Research Center, King Abdulaziz University, P.O. Box 80216, Jeddah 21589, Kingdom of Saudi Arabia 2Department of Biochemistry, All India Institute of Medical Sciences, New Delhi, 110029, India 3Department of Clinical Biochemistry, University of Kashmir, Hazratbal, Srinagar, 190006, India Abstract: Alzheimer’s disease (AD) is one of the major neurodegenerative diseases affecting almost 28 million people around the globe. It consistently remains one of the major health concerns of present world. Due to the clinical limitations like severe side effects of some synthesized drugs, alternative forms of treatments are gaining global acceptance in the treatment of AD. Neuroprotective compounds of natural origin and their synthetic derivatives exhibit promising results with minimal side effects and some of them are in their different phases of clinical trials. Alkaloids and their synthetic derivatives form one of the groups which have been used in treatment of neurodegenerative diseases like AD. We have further grouped these alkaloids into different sub groups like Indoles, piperdine and isoquinolines. Polyphenols form another important class of natural compounds used in AD management. Keywords: Alkaloids, polyphenols, Alzheimer’s disease, neuroprotective function. INTRODUCTION been proposed as one of the alternative forms of the treatment. Large number of these molecules have been Alzheimer’s disease (AD) is the most common type of reported to play significant roles in removal of deficiency of dementia and it accounts for an estimated 60 to 80 percent of neurotransmitters either by increasing their level using reported cases of dementia [1]. -
Review on Plant Antimicrobials: a Mechanistic Viewpoint Bahman Khameneh1, Milad Iranshahy2,3, Vahid Soheili1 and Bibi Sedigheh Fazly Bazzaz3*
Khameneh et al. Antimicrobial Resistance and Infection Control (2019) 8:118 https://doi.org/10.1186/s13756-019-0559-6 REVIEW Open Access Review on plant antimicrobials: a mechanistic viewpoint Bahman Khameneh1, Milad Iranshahy2,3, Vahid Soheili1 and Bibi Sedigheh Fazly Bazzaz3* Abstract Microbial resistance to classical antibiotics and its rapid progression have raised serious concern in the treatment of infectious diseases. Recently, many studies have been directed towards finding promising solutions to overcome these problems. Phytochemicals have exerted potential antibacterial activities against sensitive and resistant pathogens via different mechanisms of action. In this review, we have summarized the main antibiotic resistance mechanisms of bacteria and also discussed how phytochemicals belonging to different chemical classes could reverse the antibiotic resistance. Next to containing direct antimicrobial activities, some of them have exerted in vitro synergistic effects when being combined with conventional antibiotics. Considering these facts, it could be stated that phytochemicals represent a valuable source of bioactive compounds with potent antimicrobial activities. Keywords: Antibiotic-resistant, Antimicrobial activity, Combination therapy, Mechanism of action, Natural products, Phytochemicals Introduction bacteria [10, 12–14]. However, up to this date, the Today’s, microbial infections, resistance to antibiotic structure-activity relationships and mechanisms of action drugs, have been the biggest challenges, which threaten of natural compounds have largely remained elusive. In the health of societies. Microbial infections are responsible the present review, we have focused on describing the re- for millions of deaths every year worldwide. In 2013, 9.2 lationship between the structure of natural compounds million deaths have been reported because of infections and their possible mechanism of action. -
Herbivore Adaptation? Alkaloids Inhibiting Acetylcholinesterase As a Case 1,2, 3 MACIEJ J
CONCEPTS & THEORY Is doping of cognitive performance an anti-herbivore adaptation? Alkaloids inhibiting acetylcholinesterase as a case 1,2, 3 MACIEJ J. EJSMOND AND FREDERICK D. PROVENZA 1Institute of Environmental Sciences, Jagiellonian University, ul. Gronostajowa 7, Krakow, 30-387 Poland 2Department of Arctic Biology, The University Centre in Svalbard, Longyearbyen, N-9171 Norway 3Department of Wildland Resources, Utah State University, Logan, Utah 84322 USA Citation: Ejsmond, M. J., and F. D. Provenza. 2018. Is doping of cognitive performance an anti-herbivore adaptation? Alkaloids inhibiting acetylcholinesterase as a case. Ecosphere 9(2):e02129. 10.1002/ecs2.2129 Abstract. Historically, people who study interactions between plants and herbivores focused on the eco- logical costs and benefits of synthesizing secondary metabolites. These compounds have diverse functions including defenses against herbivores. Some plants produce alkaloids that act as acetylcholinesterase inhi- bitors, increasing both the level and duration of action of the neurotransmitter acetylcholine with potential toxic effects in insects and mammals. Yet, among a number of neuroactive plant chemicals, alkaloids that inhibit acetylcholinesterase (AIA) display nootropic activities, that is, positively affect cognition, learning, and memory in mammals. This creates a paradox: Neuroactive AIA, expected to punish herbivores, enhance cognition, learning, and memory. A prevailing view is AIA are pesticides that adversely affecting the nervous systems of herbivorous insects, and the positive influences in mammals are merely a by- product of other functions. We review literature on the behavioral ecology of diet choice, food-aversion learning, and neurophysiological actions of AIA in mammals to provide a more comprehensive view of the adaptive significance of AIA. -
Toxins and Signalling Evelyne Benoit, Françoise Goudey-Perriere, P
Toxins and Signalling Evelyne Benoit, Françoise Goudey-Perriere, P. Marchot, Denis Servent To cite this version: Evelyne Benoit, Françoise Goudey-Perriere, P. Marchot, Denis Servent. Toxins and Signalling. SFET Publications, Châtenay-Malabry, France, pp.204, 2009. hal-00738643 HAL Id: hal-00738643 https://hal.archives-ouvertes.fr/hal-00738643 Submitted on 21 May 2020 HAL is a multi-disciplinary open access L’archive ouverte pluridisciplinaire HAL, est archive for the deposit and dissemination of sci- destinée au dépôt et à la diffusion de documents entific research documents, whether they are pub- scientifiques de niveau recherche, publiés ou non, lished or not. The documents may come from émanant des établissements d’enseignement et de teaching and research institutions in France or recherche français ou étrangers, des laboratoires abroad, or from public or private research centers. publics ou privés. Collection Rencontres en Toxinologie © E. JOVER et al. TTooxxiinneess eett SSiiggnnaalliissaattiioonn -- TTooxxiinnss aanndd SSiiggnnaalllliinngg © B.J. LAVENTIE et al. Comité d’édition – Editorial committee : Evelyne BENOIT, Françoise GOUDEY-PERRIERE, Pascale MARCHOT, Denis SERVENT Société Française pour l'Etude des Toxines French Society of Toxinology Illustrations de couverture – Cover pictures : En haut – Top : Les effets intracellulaires multiples des toxines botuliques et de la toxine tétanique - The multiple intracellular effects of the BoNTs and TeNT. (Copyright Emmanuel JOVER, Fréderic DOUSSAU, Etienne LONCHAMP, Laetitia WIOLAND, Jean-Luc DUPONT, Jordi MOLGÓ, Michel POPOFF, Bernard POULAIN) En bas - Bottom : Structure tridimensionnelle de l’alpha-toxine staphylocoque - Tridimensional structure of staphylococcal alpha-toxin. (Copyright Benoit-Joseph LAVENTIE, Daniel KELLER, Emmanuel JOVER, Gilles PREVOST) Collection Rencontres en Toxinologie La collection « Rencontres en Toxinologie » est publiée à l’occasion des Colloques annuels « Rencontres en Toxinologie » organisés par la Société Française pour l’Etude des Toxines (SFET). -
Alkaloids: an Overview of Their Antibacterial, Antibiotic-Enhancing and Antivirulence Activities
International Journal of Antimicrobial Agents 44 (2014) 377–386 Contents lists available at ScienceDirect International Journal of Antimicrobial Agents j ournal homepage: http://www.elsevier.com/locate/ijantimicag Review Alkaloids: An overview of their antibacterial, antibiotic-enhancing and antivirulence activities a,∗ b c T.P. Tim Cushnie , Benjamart Cushnie , Andrew J. Lamb a Faculty of Medicine, Mahasarakham University, Khamriang, Kantarawichai, Maha Sarakham 44150, Thailand b Faculty of Pharmacy, Mahasarakham University, Khamriang, Kantarawichai, Maha Sarakham 44150, Thailand c School of Pharmacy and Life Sciences, Research Institute for Health and Wellbeing, Robert Gordon University, Riverside East, Garthdee Road, Aberdeen AB10 7GJ, UK a r t i c l e i n f o a b s t r a c t Article history: With reports of pandrug-resistant bacteria causing untreatable infections, the need for new antibac- Received 16 June 2014 terial therapies is more pressing than ever. Alkaloids are a large and structurally diverse group of Accepted 20 June 2014 compounds that have served as scaffolds for important antibacterial drugs such as metronidazole and the quinolones. In this review, we highlight other alkaloids with development potential. Natural, Keywords: semisynthetic and synthetic alkaloids of all classes are considered, looking first at those with direct Alkaloid antibacterial activity and those with antibiotic-enhancing activity. Potent examples include CJ-13,136, a Antibacterial novel actinomycete-derived quinolone alkaloid with a minimum inhibitory concentration of 0.1 ng/mL Structure–activity against Helicobacter pylori, and squalamine, a polyamine alkaloid from the dogfish shark that renders Mechanism of action Synergy Gram-negative pathogens 16- to >32-fold more susceptible to ciprofloxacin. -
Isoquinoline Alkaloids in Diets for Young Growing Pigs Improve Nutrient Digestibility and Gut Health
ISOQUINOLINE ALKALOIDS IN DIETS FOR YOUNG GROWING PIGS IMPROVE NUTRIENT DIGESTIBILITY AND GUT HEALTH BY CARLY MARIE RUNDLE THESIS Submitted in partial fulfillment of the requirements for the degree of Master of Science in Nutritional Sciences in the Graduate College of the University of Illinois at Urbana-Champaign, 2018 Urbana, Illinois Master’s Committee: Associate Professor Ryan N. Dilger, Chair Professor Hans H. Stein, Director Of Research Professor Carl M. Parsons ABSTRACT Three experiments were conducted to evaluate the effects of isoquinoline alkaloids (IQ) in corn-soybean meal (SBM) diets fed to young growing pigs on growth performance, gut health, energy and nutrient digestibility, and digestible energy (DE) and metabolizable energy (ME) concentrations in the diets. In the first experiment, 32 ileal cannulated barrows (12.19 ± 1.38 kg) were allotted to 4 diets with 8 replicate pigs per diet to determine effects of dietary inclusion of IQ on apparent ileal digestibility (AID) of amino acids (AA), crude protein (CP), starch, and acid hydrolyzed ether extract (AEE). The corn-soybean meal basal diet was supplemented with 0, 90, 180, or 360 mg IQ/kg complete feed. Diets were fed for 27 d and ileal digesta were collected on d 13 and 14 (period 1) and d 26 and 27 (period 2). Results indicated that dietary inclusion of IQ resulted in a quadratic increase (P < 0.05) in the AID of starch, Thr, Trp, Val, Pro, and Tyr in period 1. Additionally, the AID of starch was greater (P < 0.05) in period 1 than in period 2. The AID of CP, Arg, His, Ile, Leu, Met, Phe, Thr, Trp, Val, Pro, and Tyr in period 2 was greater (P < 0.05) than in period 1.