Synthesis and Evaluation of Tetrahydroprotoberberines As Dopamine Receptor Ligands
Total Page:16
File Type:pdf, Size:1020Kb
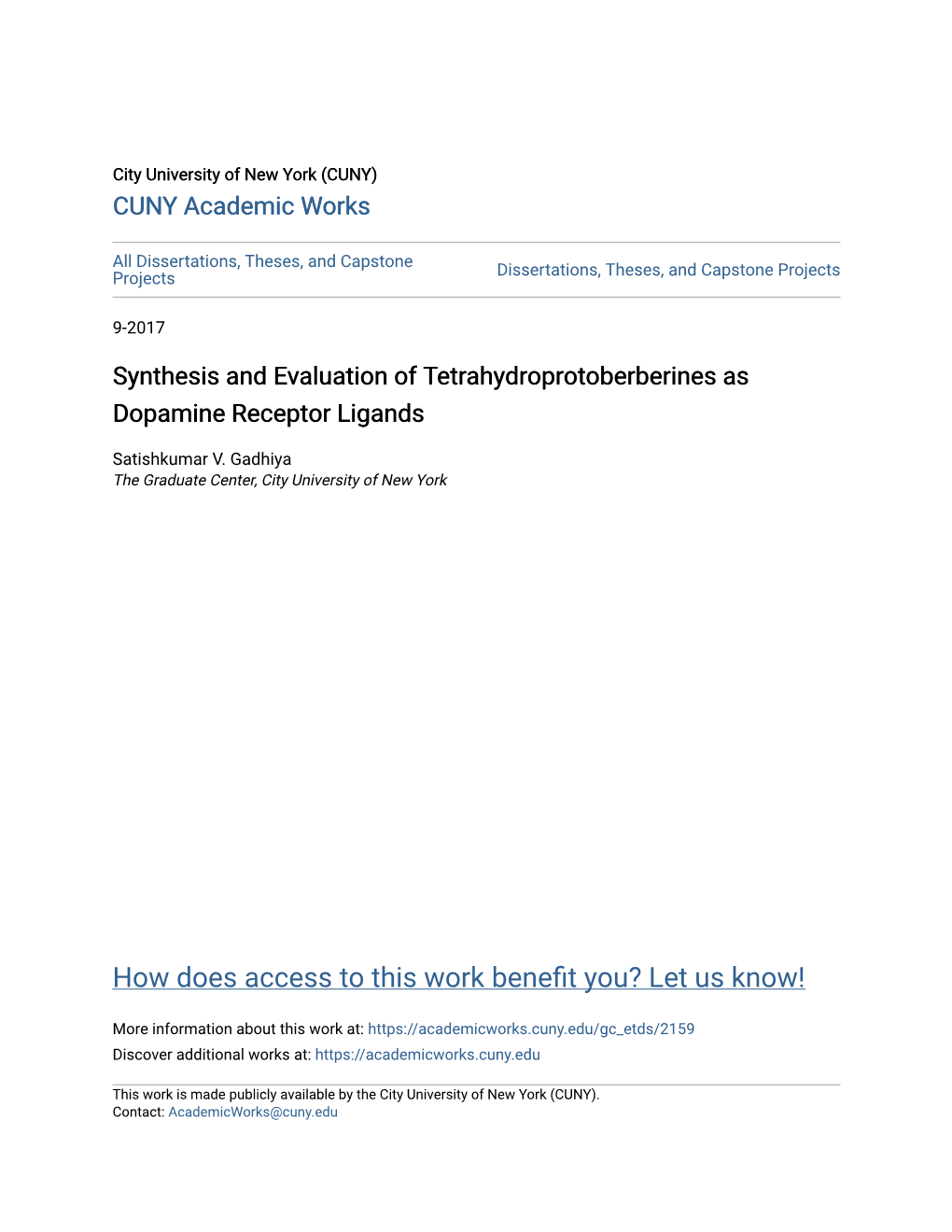
Load more
Recommended publications
-
Synaptic GABAA Receptor Clustering Without the 2 Subunit
The Journal of Neuroscience, July 30, 2014 • 34(31):10219–10233 • 10219 Cellular/Molecular ␥ Synaptic GABAA Receptor Clustering without the 2 Subunit Katalin Kerti-Szigeti, Zoltan Nusser, and X Mark D. Eyre Laboratory of Cellular Neurophysiology, Institute of Experimental Medicine, Hungarian Academy of Sciences, Budapest 1083, Hungary Rapid activation of postsynaptic GABAA receptors (GABAARs) is crucial in many neuronal functions, including the synchronization of neuronal ensembles and controlling the precise timing of action potentials. Although the ␥2 subunit is believed to be essential for the ␥ Ϫ/Ϫ postsynaptic clustering of GABAARs, synaptic currents have been detected in neurons obtained from 2 mice. To determine the role ␥ ␥ of the 2 subunit in synaptic GABAAR enrichment, we performed a spatially and temporally controlled 2 subunit deletion by injecting ␥ 77I Cre-expressing viral vectors into the neocortex of GABAAR 2 lox mice. Whole-cell recordings revealed the presence of miniature IPSCs in Cre ϩ layer 2/3 pyramidal cells (PCs) with unchanged amplitudes and rise times, but significantly prolonged decays. Such slowly decaying currents could be evoked in PCs by action potentials in presynaptic fast-spiking interneurons. Freeze-fracture replica immu- nogold labeling revealed the presence of the ␣1 and 3 subunits in perisomatic synapses of cells that lack the ␥2 subunit. Miniature IPSCs in Cre ϩ PCs were insensitive to low concentrations of flurazepam, providing a pharmacological confirmation of the lack of the ␥2 subunit. Receptors assembled from only ␣ subunits were unlikely because Zn 2ϩ did not block the synaptic currents. Pharmacological experiments indicated that the ␣␥3 receptor, rather than the ␣␦, ␣,or␣␥1 receptors, was responsible for the slowly decaying IPSCs.OurdatademonstratethepresenceofIPSCsandthesynapticenrichmentofthe␣1and3subunitsandsuggestthatthe␥3subunit ␥ is the most likely candidate for clustering GABAARs at synapses in the absence of the 2 subunit. -
Inhibition of 50-Khz Ultrasonic Vocalizations by Dopamine Receptor Subtype-Selective Agonists and Antagonists in Adult Rats
Psychopharmacology (2013) 226:589–600 DOI 10.1007/s00213-012-2931-6 ORIGINAL INVESTIGATION Inhibition of 50-kHz ultrasonic vocalizations by dopamine receptor subtype-selective agonists and antagonists in adult rats Tina Scardochio & Paul B. S. Clarke Received: 6 September 2012 /Accepted: 13 November 2012 /Published online: 29 November 2012 # Springer-Verlag Berlin Heidelberg 2012 Abstract calling. The D4 agonist and antagonist did not significantly Rationale Adult rats emit ultrasonic calls at around 22 and affect 50-kHz call rates. Twenty-two-kilohertz calls oc- 50 kHz, which are often elicited by aversive and rewarding curred infrequently under all drug conditions. stimuli, respectively. Dopamine (DA) plays a role in aspects Conclusion Following systemic drug administration, tonic of both reward and aversion. pharmacological activation of D1-like or D2-like DA recep- Objective The purpose of this study is to investigate the tors, either alone or in combination, does not appear suffi- effects of DA receptor subtype-selective agonists on 22- cient to induce 50-kHz calls. Dopaminergic transmission and 50-kHz call rates. through D1, D2, and D3 receptors appears necessary for Methods Ultrasonic calls were recorded in adult male rats spontaneous calling. that were initially screened with amphetamine to eliminate low 50-kHz callers. The remaining subjects were tested after Keywords Vocalization . Dopamine receptor . Reward . acute intraperitoneal or subcutaneous injection of the fol- Aversion . Agonist . Antagonist . Reinforcement . lowing DA receptor-selective agonists and antagonists: Behavior . D1 [D-1] . D2 [D-2] A68930 (D1-like agonist), quinpirole (D2-like agonist), PD 128907 (D3 agonist), PD 168077 (D4 agonist), SCH 39166 (D1-like antagonist), L-741,626 (D2 antagonist), Introduction NGB 2904 (D3 antagonist), and L-745,870 (D4 antagonist). -
Pharmacokinetic Interactions Between Herbal Medicines and Drugs: Their Mechanisms and Clinical Relevance
life Review Pharmacokinetic Interactions between Herbal Medicines and Drugs: Their Mechanisms and Clinical Relevance Laura Rombolà 1 , Damiana Scuteri 1,2 , Straface Marilisa 1, Chizuko Watanabe 3, Luigi Antonio Morrone 1, Giacinto Bagetta 1,2,* and Maria Tiziana Corasaniti 4 1 Preclinical and Translational Pharmacology, Department of Pharmacy, Health and Nutritional Sciences, Section of Preclinical and Translational Pharmacology, University of Calabria, 87036 Rende, Italy; [email protected] (L.R.); [email protected] (D.S.); [email protected] (S.M.); [email protected] (L.A.M.) 2 Pharmacotechnology Documentation and Transfer Unit, Preclinical and Translational Pharmacology, Department of Pharmacy, Health and Nutritional Sciences, University of Calabria, 87036 Rende, Italy 3 Department of Physiology and Anatomy, Tohoku Pharmaceutical University, 981-8558 Sendai, Japan; [email protected] 4 School of Hospital Pharmacy, University “Magna Graecia” of Catanzaro and Department of Health Sciences, University “Magna Graecia” of Catanzaro, 88100 Catanzaro, Italy; [email protected] * Correspondence: [email protected]; Tel.: +39-0984-493462 Received: 28 May 2020; Accepted: 30 June 2020; Published: 4 July 2020 Abstract: The therapeutic efficacy of a drug or its unexpected unwanted side effects may depend on the concurrent use of a medicinal plant. In particular, constituents in the medicinal plant extracts may influence drug bioavailability, metabolism and half-life, leading to drug toxicity or failure to obtain a therapeutic response. This narrative review focuses on clinical studies improving knowledge on the ability of selected herbal medicines to influence the pharmacokinetics of co-administered drugs. Moreover, in vitro studies are useful to anticipate potential herbal medicine-drug interactions. -
Sex Differences in Nicotine-Conditioned Hyperactivity in a Model of Dopamine D2 Receptor Priming: Roles of Dopamine D2 and D3 Receptor Subtypes
East Tennessee State University Digital Commons @ East Tennessee State University Electronic Theses and Dissertations Student Works 8-2008 Sex Differences in Nicotine-Conditioned Hyperactivity in a Model of Dopamine D2 Receptor Priming: Roles of Dopamine D2 and D3 Receptor Subtypes. Ashley Brianna Sheppard East Tennessee State University Follow this and additional works at: https://dc.etsu.edu/etd Part of the Hormones, Hormone Substitutes, and Hormone Antagonists Commons Recommended Citation Sheppard, Ashley Brianna, "Sex Differences in Nicotine-Conditioned Hyperactivity in a Model of Dopamine D2 Receptor Priming: Roles of Dopamine D2 and D3 Receptor Subtypes." (2008). Electronic Theses and Dissertations. Paper 1978. https://dc.etsu.edu/etd/ 1978 This Thesis - Open Access is brought to you for free and open access by the Student Works at Digital Commons @ East Tennessee State University. It has been accepted for inclusion in Electronic Theses and Dissertations by an authorized administrator of Digital Commons @ East Tennessee State University. For more information, please contact [email protected]. Sex Differences in Nicotine-conditioned Hyperactivity in a Model of Dopamine D2 Receptor Priming: Roles of Dopamine D2 and D3 Receptor Subtypes A thesis presented to the faculty of the Department of Psychology East Tennessee State University In partial fulfillment of the requirements for the degree of Master of Arts in Psychology by Ashley Brianna Sheppard August 2008 Russell Brown, PhD., Chair Michael Woodruff, PhD., Committee member Otto Zinser, -
“Biosynthesis of Morphine in Mammals”
“Biosynthesis of Morphine in Mammals” D i s s e r t a t i o n zur Erlangung des akademischen Grades Doctor rerum naturalium (Dr. rer. nat.) vorgelegt der Naturwissenschaftlichen Fakultät I Biowissenschaften der Martin-Luther-Universität Halle-Wittenberg von Frau Nadja Grobe geb. am 21.08.1981 in Querfurt Gutachter /in 1. 2. 3. Halle (Saale), Table of Contents I INTRODUCTION ........................................................................................................1 II MATERIAL & METHODS ........................................................................................ 10 1 Animal Tissue ....................................................................................................... 10 2 Chemicals and Enzymes ....................................................................................... 10 3 Bacteria and Vectors ............................................................................................ 10 4 Instruments ........................................................................................................... 11 5 Synthesis ................................................................................................................ 12 5.1 Preparation of DOPAL from Epinephrine (according to DUNCAN 1975) ................. 12 5.2 Synthesis of (R)-Norlaudanosoline*HBr ................................................................. 12 5.3 Synthesis of [7D]-Salutaridinol and [7D]-epi-Salutaridinol ..................................... 13 6 Application Experiments ..................................................................................... -
Enantioenriched Positive Allosteric Modulators Display Distinct Pharmacology at the Dopamine D1 Receptor
molecules Article Enantioenriched Positive Allosteric Modulators Display Distinct Pharmacology at the Dopamine D1 Receptor Tim J. Fyfe 1, Peter J. Scammells 1, J. Robert Lane 2,3,* and Ben Capuano 1,* 1 Medicinal Chemistry, Monash Institute of Pharmaceutical Sciences, Monash University, 381 Royal Parade, Parkville, VIC 3052, Australia; tim.fyfe@griffithhack.com (T.J.F.); [email protected] (P.J.S.) 2 Drug Discovery Biology, Monash Institute of Pharmaceutical Sciences, Monash University, 381 Royal Parade, Parkville, VIC 3052, Australia 3 School of Life Sciences, Queen’s Medical Centre, University of Nottingham, Nottingham NG7 2UH, UK * Correspondence: [email protected] (J.R.L.); [email protected] (B.C.) Abstract: (1) Background: Two first-in-class racemic dopamine D1 receptor (D1R) positive allosteric modulator (PAM) chemotypes (1 and 2) were identified from a high-throughput screen. In particular, due to its selectivity for the D1R and reported lack of intrinsic activity, compound 2 shows promise as a starting point toward the development of small molecule allosteric modulators to ameliorate the cognitive deficits associated with some neuropsychiatric disease states; (2) Methods: Herein, we describe the enantioenrichment of optical isomers of 2 using chiral auxiliaries derived from (R)- and (S)-3-hydroxy-4,4-dimethyldihydrofuran-2(3H)-one (D- and L-pantolactone, respectively); (3) Results: We confirm both the racemate and enantiomers of 2 are active and selective for the D1R, but that the respective stereoisomers show a significant difference in their affinity and magnitude of positive allosteric cooperativity with dopamine; (4) Conclusions: These data warrant further investigation Citation: Fyfe, T.J.; Scammells, P.J.; of asymmetric syntheses of optically pure analogues of 2 for the development of D1R PAMs with Lane, J.R.; Capuano, B. -
(19) United States (12) Patent Application Publication (10) Pub
US 20130289061A1 (19) United States (12) Patent Application Publication (10) Pub. No.: US 2013/0289061 A1 Bhide et al. (43) Pub. Date: Oct. 31, 2013 (54) METHODS AND COMPOSITIONS TO Publication Classi?cation PREVENT ADDICTION (51) Int. Cl. (71) Applicant: The General Hospital Corporation, A61K 31/485 (2006-01) Boston’ MA (Us) A61K 31/4458 (2006.01) (52) U.S. Cl. (72) Inventors: Pradeep G. Bhide; Peabody, MA (US); CPC """"" " A61K31/485 (201301); ‘4161223011? Jmm‘“ Zhu’ Ansm’ MA. (Us); USPC ......... .. 514/282; 514/317; 514/654; 514/618; Thomas J. Spencer; Carhsle; MA (US); 514/279 Joseph Biederman; Brookline; MA (Us) (57) ABSTRACT Disclosed herein is a method of reducing or preventing the development of aversion to a CNS stimulant in a subject (21) App1_ NO_; 13/924,815 comprising; administering a therapeutic amount of the neu rological stimulant and administering an antagonist of the kappa opioid receptor; to thereby reduce or prevent the devel - . opment of aversion to the CNS stimulant in the subject. Also (22) Flled' Jun‘ 24’ 2013 disclosed is a method of reducing or preventing the develop ment of addiction to a CNS stimulant in a subj ect; comprising; _ _ administering the CNS stimulant and administering a mu Related U‘s‘ Apphcatlon Data opioid receptor antagonist to thereby reduce or prevent the (63) Continuation of application NO 13/389,959, ?led on development of addiction to the CNS stimulant in the subject. Apt 27’ 2012’ ?led as application NO_ PCT/US2010/ Also disclosed are pharmaceutical compositions comprising 045486 on Aug' 13 2010' a central nervous system stimulant and an opioid receptor ’ antagonist. -
Coptis Japonica</Emphasis>
Plant Cell Reports (1988) 7:1-4 Plant Cell Reports © Springer-Verlag 1988 Alternative final steps in berberine biosynthesis in Coptisjaponica cell cultures E. Galneder 1 M. Rueffer 1, G. Wanner 1, 2, M. Tabata 1, 3, and M. H. Zenk 1 1 Lehrstuhl tar Pharmazeutische Biologie der Universitfit Mfinchen, Karlstrasse 29, D-8000 Mt~nchen 2, Federal Republic of Germany 2 Botanisches Institut der Universitfit Miinchen, Menzinger Strasse 67, D-8000 Manchen 19, Federal Republic of Germany 3 Faculty of Pharmaceutical Sciences, Kyoto University, Kyoto 606, Japan Received October 30, 1987 - Communicated by K. Hahlbrock ABSTRACT oxidase (STOX) reported from our laboratory (Amann et al., 1984) in that the Coptis enzyme dehydrogenated In Coptis japonica cell cultures an alternative path- only (S)-canadine while other tetrahydroprotober- way has been discovered which leads from (S)-tetra- berines were reported to be inactive. In further hydrocolumbamine via (S)-canadine to berberine. The contrast to the STOX enzyme, their enzyme did not two enzymes involved have been partially purified. produce hydrogen peroxide but rather H20 as one of (S)-Tetrahydrocolumbamine is stereospecifically the reaction products. Our analysis of the Coptis transformed into (S)-canadine under formation of the system reported here led to the surprising result methylenedioxy bridge in ring A. This new enzyme was that the terminal two steps in the biosynthesis of named (S)-canad/ne synthase. (S)-Canadine in turn is berberine in 8erberis and Coptis are biochemically stereospecifically dehydrogenated to berberine by an completely different while similar at the cytological oxidase, (S)-canadine oxidase (COX), which was level. -
NINDS Custom Collection II
ACACETIN ACEBUTOLOL HYDROCHLORIDE ACECLIDINE HYDROCHLORIDE ACEMETACIN ACETAMINOPHEN ACETAMINOSALOL ACETANILIDE ACETARSOL ACETAZOLAMIDE ACETOHYDROXAMIC ACID ACETRIAZOIC ACID ACETYL TYROSINE ETHYL ESTER ACETYLCARNITINE ACETYLCHOLINE ACETYLCYSTEINE ACETYLGLUCOSAMINE ACETYLGLUTAMIC ACID ACETYL-L-LEUCINE ACETYLPHENYLALANINE ACETYLSEROTONIN ACETYLTRYPTOPHAN ACEXAMIC ACID ACIVICIN ACLACINOMYCIN A1 ACONITINE ACRIFLAVINIUM HYDROCHLORIDE ACRISORCIN ACTINONIN ACYCLOVIR ADENOSINE PHOSPHATE ADENOSINE ADRENALINE BITARTRATE AESCULIN AJMALINE AKLAVINE HYDROCHLORIDE ALANYL-dl-LEUCINE ALANYL-dl-PHENYLALANINE ALAPROCLATE ALBENDAZOLE ALBUTEROL ALEXIDINE HYDROCHLORIDE ALLANTOIN ALLOPURINOL ALMOTRIPTAN ALOIN ALPRENOLOL ALTRETAMINE ALVERINE CITRATE AMANTADINE HYDROCHLORIDE AMBROXOL HYDROCHLORIDE AMCINONIDE AMIKACIN SULFATE AMILORIDE HYDROCHLORIDE 3-AMINOBENZAMIDE gamma-AMINOBUTYRIC ACID AMINOCAPROIC ACID N- (2-AMINOETHYL)-4-CHLOROBENZAMIDE (RO-16-6491) AMINOGLUTETHIMIDE AMINOHIPPURIC ACID AMINOHYDROXYBUTYRIC ACID AMINOLEVULINIC ACID HYDROCHLORIDE AMINOPHENAZONE 3-AMINOPROPANESULPHONIC ACID AMINOPYRIDINE 9-AMINO-1,2,3,4-TETRAHYDROACRIDINE HYDROCHLORIDE AMINOTHIAZOLE AMIODARONE HYDROCHLORIDE AMIPRILOSE AMITRIPTYLINE HYDROCHLORIDE AMLODIPINE BESYLATE AMODIAQUINE DIHYDROCHLORIDE AMOXEPINE AMOXICILLIN AMPICILLIN SODIUM AMPROLIUM AMRINONE AMYGDALIN ANABASAMINE HYDROCHLORIDE ANABASINE HYDROCHLORIDE ANCITABINE HYDROCHLORIDE ANDROSTERONE SODIUM SULFATE ANIRACETAM ANISINDIONE ANISODAMINE ANISOMYCIN ANTAZOLINE PHOSPHATE ANTHRALIN ANTIMYCIN A (A1 shown) ANTIPYRINE APHYLLIC -
Pharmacological Stimulation of Brain Dopamine D3 Receptors Induced
ABSTRACT Pharmacological stimulation of brain dopamine D3 receptors induced ejaculation in anaesthetised rats # 163 Pierre Clément 1, Jacques Bernabé 1, Laurent Alexandre 1, François Giuliano 1,2 * 1PELVIPHARM Laboratories, Gif-sur-Yvette, France 2Raymond Poincaré Hospital, Neuro-Urology Unit, Garches, France * e-mail address: [email protected] ABSTRACT INTRODUCTION & OBJECTIVE RESULTS Introduction and Objective : We have previously found that intracerebroventricular (i.c.v.) Ejaculation consists in two distinct and successive phases i.e. emission and expulsion with the latter caused Figure 1 . Sample of EMG recording of the injection of the non selective dopamine D2/D3 receptors agonist by rhythmic contractions of pelvic floor striated muscles; the primary role being played by bulbospongiosus 7-OH-DPAT i.c.v. bulbospongiosus (BS) muscles obtained in quinelorane induced rhythmic (BS) muscles (Gerstenberg et al., 1990). (1 µg) anaesthetised rats after i.c.v. delivery of 7- contractions of bulbospongiosus 10 s (BS) muscles, which is key for OH-DPAT (1 µg). expelling semen out the urethra, in anaesthetised rats. In the present We have previously reported that the dopamine D2-like receptor agonist quinelorane delivered in cerebral A magnification of the tracing of BS-EMG is study we aimed at demonstrating ventricle was capable to elicit rhythmic BS muscles contractions in anaesthetised rats (Clement et al., 2006; displayed in the inset and allows to see the which of these dopamine receptor subtypes is involved in triggering BS EAU 2006, poster #164). In addition it has been shown that the preferential D3 receptor agonist 7-OH-DPAT V unitary events (i.e. -
The Phytochemistry of Cherokee Aromatic Medicinal Plants
medicines Review The Phytochemistry of Cherokee Aromatic Medicinal Plants William N. Setzer 1,2 1 Department of Chemistry, University of Alabama in Huntsville, Huntsville, AL 35899, USA; [email protected]; Tel.: +1-256-824-6519 2 Aromatic Plant Research Center, 230 N 1200 E, Suite 102, Lehi, UT 84043, USA Received: 25 October 2018; Accepted: 8 November 2018; Published: 12 November 2018 Abstract: Background: Native Americans have had a rich ethnobotanical heritage for treating diseases, ailments, and injuries. Cherokee traditional medicine has provided numerous aromatic and medicinal plants that not only were used by the Cherokee people, but were also adopted for use by European settlers in North America. Methods: The aim of this review was to examine the Cherokee ethnobotanical literature and the published phytochemical investigations on Cherokee medicinal plants and to correlate phytochemical constituents with traditional uses and biological activities. Results: Several Cherokee medicinal plants are still in use today as herbal medicines, including, for example, yarrow (Achillea millefolium), black cohosh (Cimicifuga racemosa), American ginseng (Panax quinquefolius), and blue skullcap (Scutellaria lateriflora). This review presents a summary of the traditional uses, phytochemical constituents, and biological activities of Cherokee aromatic and medicinal plants. Conclusions: The list is not complete, however, as there is still much work needed in phytochemical investigation and pharmacological evaluation of many traditional herbal medicines. Keywords: Cherokee; Native American; traditional herbal medicine; chemical constituents; pharmacology 1. Introduction Natural products have been an important source of medicinal agents throughout history and modern medicine continues to rely on traditional knowledge for treatment of human maladies [1]. Traditional medicines such as Traditional Chinese Medicine [2], Ayurvedic [3], and medicinal plants from Latin America [4] have proven to be rich resources of biologically active compounds and potential new drugs. -
L-Tetrahydropalmatine Reduces Nicotine Self-Administration and Reinstatement in Rats
Faison et al. BMC Pharmacology and Toxicology (2016) 17:49 DOI 10.1186/s40360-016-0093-6 RESEARCH ARTICLE Open Access l-tetrahydropalmatine reduces nicotine self- administration and reinstatement in rats Shamia L. Faison1,2, Charles W. Schindler2, Steven R. Goldberg2ˆ and Jia Bei Wang1* Abstract Background: The negative consequences of nicotine use are well known and documented, however, abstaining from nicotine use and achieving abstinence poses a major challenge for the majority of nicotine users trying to quit. l-Tetrahydropalmatine (l-THP), a compound extracted from the Chinese herb Corydalis, displayed utility in the treatment of cocaine and heroin addiction via reduction of drug-intake and relapse. The present study examined the effects of l-THP on abuse-related effects of nicotine. Methods: Self-administration and reinstatement testing was conducted. Rats trained to self-administer nicotine (0.03 mg/kg/injection) under a fixed-ratio 5 schedule (FR5) of reinforcement were pretreated with l-THP (3 or 5 mg/kg), varenicline (1 mg/kg), bupropion (40 mg/kg), or saline before daily 2-h sessions. Locomotor, food, and microdialysis assays were also conducted in separate rats. Results: l-THP significantly reduced nicotine self-administration (SA). l-THP’s effect was more pronounced than the effect of varenicline and similar to the effect of bupropion. In reinstatement testing, animals were pretreated with the same compounds, challenged with nicotine (0.3 mg/kg, s.c.), and reintroduced to pre-extinction conditions. l-THP blocked reinstatement of nicotine seeking more effectively than either varenicline or bupropion. Locomotor data revealed that therapeutic doses of l-THP had no inhibitory effects on ambulatory ability and that l-THP (3 and 5 mg/kg) significantly blocked nicotine induced hyperactivity when administered before nicotine.