An Idealized Numerical Study of Tropical Cyclogenesis and Evolution at the Equator
Total Page:16
File Type:pdf, Size:1020Kb
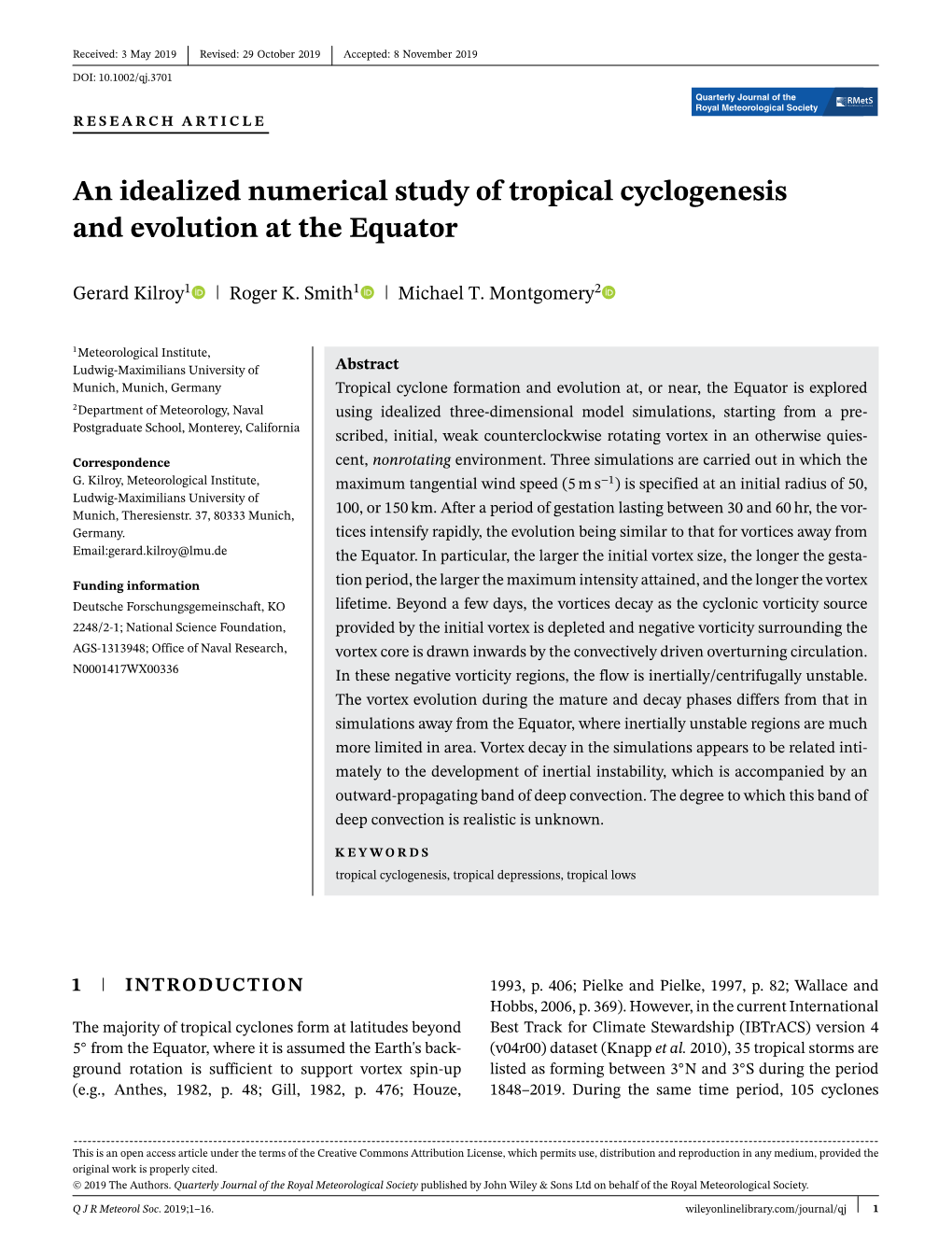
Load more
Recommended publications
-
Annual Weather/Climate Data Summary 2010 Pacific Island Network
National Park Service U.S. Department of the Interior Natural Resource Stewardship and Science Annual Weather/Climate Data Summary 2010 Pacific Island Network Natural Resource Data Series NPS/PACN/NRDS—2012/273 ON THE COVER Sunset at American Memorial Park (AMME), Saipan, Commonwealth of the Northern Mariana Islands. Photograph courtesy of National Park Service staff. Annual Weather/Climate Data Summary 2010 Pacific Island Network Natural Resource Data Series NPS/PACN/NRDS—2012/273 Tonnie L. C. Casey National Park Service Inventory and Monitoring P.O. Box 52 Hawaii National Park, HI 96718 April 2012 U.S. Department of the Interior National Park Service Natural Resource Stewardship and Science Fort Collins, Colorado The National Park Service, Natural Resource Stewardship and Science office in Fort Collins, Colorado publishes a range of reports that address natural resource topics of interest and applicability to a broad audience in the National Park Service and others in natural resource management, including scientists, conservation and environmental constituencies, and the public. The Natural Resource Data Series is intended for the timely release of basic data sets and data summaries. Care has been taken to assure accuracy of raw data values, but a thorough analysis and interpretation of the data has not been completed. Consequently, the initial analyses of data in this report are provisional and subject to change. All manuscripts in the series receive the appropriate level of peer review to ensure that the information is scientifically credible, technically accurate, appropriately written for the intended audience, and designed and published in a professional manner. Data in this report were collected and analyzed using methods based on established, peer- reviewed protocols and were analyzed and interpreted within the guidelines of the protocols. -
Cranking up the Intensity: Climate Change and Extreme Weather Events
CRANKING UP THE INTENSITY: CLIMATE CHANGE AND EXTREME WEATHER EVENTS CLIMATECOUNCIL.ORG.AU Thank you for supporting the Climate Council. The Climate Council is an independent, crowd-funded organisation providing quality information on climate change to the Australian public. Published by the Climate Council of Australia Limited ISBN: 978-1-925573-14-5 (print) 978-1-925573-15-2 (web) © Climate Council of Australia Ltd 2017 This work is copyright the Climate Council of Australia Ltd. All material Professor Will Steffen contained in this work is copyright the Climate Council of Australia Ltd Climate Councillor except where a third party source is indicated. Climate Council of Australia Ltd copyright material is licensed under the Creative Commons Attribution 3.0 Australia License. To view a copy of this license visit http://creativecommons.org.au. You are free to copy, communicate and adapt the Climate Council of Australia Ltd copyright material so long as you attribute the Climate Council of Australia Ltd and the authors in the following manner: Cranking up the Intensity: Climate Change and Extreme Weather Events by Prof. Lesley Hughes Professor Will Steffen, Professor Lesley Hughes, Dr David Alexander and Dr Climate Councillor Martin Rice. The authors would like to acknowledge Prof. David Bowman (University of Tasmania), Dr. Kathleen McInnes (CSIRO) and Dr. Sarah Perkins-Kirkpatrick (University of New South Wales) for kindly reviewing sections of this report. We would also like to thank Sally MacDonald, Kylie Malone and Dylan Pursche for their assistance in preparing the report. Dr David Alexander Researcher, — Climate Council Image credit: Cover Photo “All of this sand belongs on the beach to the right” by Flickr user Rob and Stephanie Levy licensed under CC BY 2.0. -
Pacific ENSO Update Page 16
2nd Quarter, 2016 Vol. 22, No. 2 ISSUED: May 23rd, 2016 Providing Information on Climate Variability in the U.S.-Affiliated Pacific Islands for the Past 20 Years. http://www.weather.gov/peac CURRENT CONDITIONS The epic El Niño of 2015/16 is now in its post-Peak phase In response to the impact of drought conditions on water sup- (Figure 1), with the CPC’s Oceanic Niño Index now beginning ply, local governments have issued proclamations concerning its fall away from its peak value reached in December 2015 drought: the governments of Palau and of the FSM have de- (Figure 2). Very dry conditions have developed over the past clared drought emergencies for portions of their jurisdictions, few months across much of Micronesia and into Hawaii (Figures and the government of the Republic of the Marshall Islands 23 and 24). Prolonged and widespread dry conditions are typi- has gone even further with a declaration of drought disaster. cal during the post-Peak phase of a strong El Niño. The follow- On 27 April, President Obama issued Presidential Declaration ing records for low rainfall have been set (compiled from NOAA NCEI): for the Republic of the Marshalls Islands in response to earlier RMI request. With very dry conditions ongoing, the nature (1) Koror, Palau — driest Oct-Mar, driest Apr-Mar; and extent of impacts is still being gathered and assessed. (2) Yap Island — driest Oct-Mar; Substantial draw-down and degradation of municipal water (3) Pohnpei Island — 3rd driest Oct-Mar, 4th driest Apr-Mar; supplies is widespread, streamflow on high islands is very (4) Nukuoro (Pohnpei State) — driest Apr-Mar, 3rd driest Oct-Mar; (5) Ulithi (Yap State) — 2nd driest Oct-Mar, driest March; low, and vegetation has suffered (yellowing, wilting, and de- (6) Woleai (Yap State) — driest Oct-Mar, driest Apr-Mar; struction by wildfire). -
The Tropics—H
4. THE TROPICS—H. J. Diamond and C. J. Schreck, Eds. b. ENSO and the Tropical Pacific—G. Bell, M. L’Heureux, a. Overview—H. J. Diamond and C. J. Schreck and M. S. Halpert In 2016 the Tropics were dominated by a transition The El Niño–Southern Oscillation (ENSO) is a from El Niño to La Niña. The year started with an on- coupled ocean–atmosphere climate phenomenon going El Niño that proved to be one of the strongest in over the tropical Pacific Ocean, with opposing phases the 1950–2016 record. After its peak in late 2015/early called El Niño and La Niña. For historical purposes, 2016, this El Niño weakened until it was officially NOAA’s Climate Prediction Center (CPC) classifies declared to have ended in May. El Niño–Southern and assesses the strength and duration of El Niño and Oscillation conditions were briefly neutral during the La Niña using the Oceanic Niño index (ONI; shown transition period before a weak La Niña developed for 2015 and 2016 in Fig. 4.1). The ONI is the 3-month in October. A negative Indian Ocean dipole is com- average of sea surface temperature (SST) anomalies monly associated with the transition from El Niño in the Niño-3.4 region (5°N–5°S, 170°–120°W; black to La Niña, and 2016 was one of the most strongly box in Fig. 4.3e) calculated as the departure from the negative on record. The transition was also reflected 1981–2010 base period. El Niño is classified when in the dichotomy of precipitation patterns between the ONI is at or warmer than 0.5°C for at least five the first and second halves of the year. -
A Report on All Cyclones That Formed in 2016, with Detailed Season Statistics and Records That Were Achieved Worldwide This Year
A report on all cyclones that formed in 2016, with detailed season statistics and records that were achieved worldwide this year. Compiled by Nathan Foy at Force Thirteen, December 2016, January 2017 Direct contact: [email protected] See last page of document for more contact details Cover photo: International Space Station photo of Super Typhoon Nepartak on July 7, 2016 Below: Himawari-8 visible image of Super Typhoon Haima on October 18, 2016 Contents 1. Background 3 2. The 2016 Datasheet 4 2.1 Peak Intensities 4 2.2 Amount of Landfalls and Nations Affected 7 2.3 Fatalities, Injuries, and Missing persons 10 2.4 Monetary damages 12 2.5 Buildings damaged and destroyed 13 2.6 Evacuees 15 2.7 Timeline 16 3. Notable Storms of 2016 22 3.1 Hurricane Alex 23 3.2 Cyclone Winston 24 3.3 Cyclone Fantala 25 3.4 June system in the Gulf of Mexico (“Colin”) 26 3.5 Super Typhoon Nepartak 27 3.6 Super Typhoon Meranti 28 3.7 Subtropical Storm in the Bay of Biscay 29 3.8 Hurricane Karl 30 3.9 Hurricane Matthew 31 3.10 Tropical Storm Tina 33 3.11 Hurricane Otto 34 4. 2016 Storm Records 35 4.1 Intensity and Longevity 36 4.2 Activity Records 39 4.3 Landfall Records 41 4.4 Eye and Size Records 42 4.5 Intensification Rate 43 4.6 Damages 44 5. Force Thirteen during 2016 45 5.1 Forecasting critique and storm coverage 46 5.2 Viewing statistics 47 6. Long Term Trends 48 7. -
National Hurricane Operations Plan FCM-P12-2010
U.S. DEPARTMENT OF COMMERCE/ National Oceanic and Atmospheric Administration OFFICE OF THE FEDERAL COORDINATOR FOR METEOROLOGICAL SERVICES AND SUPPORTING RESEARCH National Hurricane Operations Plan FCM-P12-2010 Hurricane Bill - 19 August 2009 Washington, DC May 2010 THE FEDERAL COMMITTEE FOR METEOROLOGICAL SERVICES AND SUPPORTING RESEARCH (FCMSSR) DR. JANE LUBCHENCO DR. RANDOLPH LYON Chairman, Department of Commerce Office of Management and Budget MS. SHERE ABBOTT MS. VICTORIA COX Office of Science and Technology Policy Department of Transportation DR. RAYMOND MOTHA MR. EDWARD CONNOR (Acting) Department of Agriculture Federal Emergency Management Agency Department of Homeland Security DR. JOHN (JACK) L. HAYES Department of Commerce DR. EDWARD WEILER National Aeronautics and Space MR. ALAN SHAFFER Administration Department of Defense DR. TIM KILLEEN DR. ANNA PALMISANO National Science Foundation Department of Energy MR. PAUL MISENCIK MR. KEVIN (SPANKY) KIRSCH National Transportation Safety Board Science and Technology Directorate Department of Homeland Security MR. JAMES LYONS U.S. Nuclear Regulatory Commission DR. HERBERT FROST Department of the Interior DR. LAWRENCE REITER Environmental Protection Agency MR. KENNETH HODGKINS Department of State MR. SAMUEL P. WILLIAMSON Federal Coordinator MR. MICHAEL BABCOCK, Secretariat Office of the Federal Coordinator for Meteorological Services and Supporting Research THE INTERDEPARTMENTAL COMMITTEE FOR METEOROLOGICAL SERVICES AND SUPPORTING RESEARCH (ICMSSR) MR. SAMUEL P. WILLIAMSON, Chairman MR. BARRY SCOTT Federal Coordinator Federal Aviation Administration Department of Transportation MR. THOMAS PUTERBAUGH Department of Agriculture DR. JONATHAN M. BERKSON United States Coast Guard DR. JOHN (JACK) L. HAYES Department of Homeland Security Department of Commerce DR. DEBORAH LAWRENCE RADM DAVID TITLEY, USN Department of State United States Navy Department of Defense DR. -
HURRICANE PALI (CP012016) 7 – 14 January 2016
CENTRAL PACIFIC HURRICANE CENTER TROPICAL CYCLONE REPORT HURRICANE PALI (CP012016) 7 – 14 January 2016 Derek Wroe and Sam Houston Central Pacific Hurricane Center 13 December 2018 GOES WEST IMAGE OF HURRICANE PALI NEAR TIME OF MAXIMIUM INTENSITY ON 12 JANUARY 2016 Within the central North Pacific basin, Pali was the earliest tropical cyclone ever observed to form in a calendar year. Pali developed at an extraordinarily low latitude and spent its entire life cycle within the deep tropics just east of the International Date Line. Pali peaked as a category 2 hurricane (on the Saffir-Simpson Hurricane Wind Scale). Hurricane Pali 2 Hurricane Pali 7 – 14 JANUARY 2016 SYNOPTIC HISTORY Although Pali developed in early 2016, its formation at extraordinarily low latitude arguably occurred as an extension of the record-breaking 2015 central North Pacific tropical cyclone season. One of the strongest El Niño events ever observed created an environment conducive for the highly active tropical cyclone season. As El Niño peaked in late December 2015 and early January 2016, a strong westerly wind burst, a classic El Niño signature, occurred along the equator across the western and central Pacific. During late December, this long-lived westerly wind burst spawned Tropical Depression Nine-C in the central North Pacific and its twin, Tropical Cyclone Ula, in the central South Pacific. Tropical Depression Nine-C quickly dissipated as the New Year was ushered in. However, a low-latitude, west-to-east oriented surface trough remained across the western and central North Pacific between 01N and 03N latitude as far east as 155W longitude. -
National Hurricane Operations Plan
U.S. DEPARTMENT OF COMMERCE/ National Oceanic and Atmospheric Administration OFFICE OF THE FEDERAL COORDINATOR FOR METEOROLOGICAL SERVICES AND SUPPORTING RESEARCH National Hurricane Operations Plan FCM-P12-2001 Washington, DC Hurricane Keith - 1 October 2000 May 2001 THE FEDERAL COMMITTEE FOR METEOROLOGICAL SERVICES AND SUPPORTING RESEARCH (FCMSSR) MR. SCOTT B. GUDES, Chairman MR. MONTE BELGER Department of Commerce Department of Transportation DR. ROSINA BIERBAUM MS. MARGARET LAWLESS (Acting) Office of Science and Technology Policy Federal Emergency Management Agency DR. RAYMOND MOTHA DR. GHASSEM R. ASRAR Department of Agriculture National Aeronautics and Space Administration MR. JOHN J. KELLY, JR. Department of Commerce DR. MARGARET S. LEINEN National Science Foundation CAPT FRANK GARCIA, USN Department of Defense MR. PAUL MISENCIK National Transportation Safety Board DR. ARISTIDES PATRINOS Department of Energy MR. ROY P. ZIMMERMAN U.S. Nuclear Regulatory Commission DR. ROBERT M. HIRSCH Department of the Interior DR. JOEL SCHERAGA (Acting) Environmental Protection Agency MR. RALPH BRAIBANTI Department of State MR. SAMUEL P. WILLIAMSON Federal Coordinator MR. RANDOLPH LYON Office of Management and Budget MR. JAMES B. HARRISON, Executive Secretary Office of the Federal Coordinator for Meteorological Services and Supporting Research THE INTERDEPARTMENTAL COMMITTEE FOR METEOROLOGICAL SERVICES AND SUPPORTING RESEARCH (ICMSSR) MR. SAMUEL P. WILLIAMSON, Chairman DR. JONATHAN M. BERKSON Federal Coordinator United States Coast Guard Department of Transportation DR. RAYMOND MOTHA Department of Agriculture DR. JOEL SCHERAGA (Acting) Environmental Protection Agency MR. JOHN E. JONES, JR. Department of Commerce MR. JOHN GAMBEL Federal Emergency Management Agency CAPT FRANK GARCIA, USN Department of Defense DR. RAMESH KAKAR National Aeronautics and Space MR. -
2017 National Hurricane Operations Plan, with Change 1
U.S. DEPARTMENT OF COMMERCE/ National Oceanic and Atmospheric Administration OFFICE OF THE FEDERAL COORDINATOR FOR METEOROLOGICAL SERVICES AND SUPPORTING RESEARCH National Hurricane Operations Plan FCM-P12-2017 Washington, DC May 2017 Front cover: Hurricane Matthew at 2115 GMT September 30, 2016, undergoing rapid intensification on the way to becoming the first Category 5 Atlantic storm in more than nine years and the southernmost Category 5 storm on record (28 miles south of Hurricane Ivan). Matthew intensified from a tropical storm at 1500 GMT on September 29th to Category 5 at 0300 GMT on October 1st, with the sustained winds increasing from 70 to 160 mph and the central pressure decreasing from 996 to 941 hPa. Image courtesy of NASA/NOAA GOES Project. FEDERAL COORDINATOR FOR METEOROLOGICAL SERVICES AND SUPPORTING RESEARCH 1325 East-West Highway, SSMC2 Silver Spring, Maryland 20910 301-628-0112 http://www.ofcm.gov/ NATIONAL HURRICANE OPERATIONS PLAN http://www.ofcm.gov/publications/nhop/FCM-P12-2017.pdf FCM-P12-2017 Washington, D.C. May 2017 CHANGE AND REVIEW LOG Use this page to record changes and notices of reviews. Change Page Numbers Date Posted Initials Number (mm/dd/yyyy) 1. I-2, L-1 5/4/2017 JS 2. 3. 4. 5. Changes are indicated by a vertical line in the margin next to the change or by shading and strikeouts. No. Review Date Comments Initials (mm/dd/yyyy) 1. 2. 3. 4. 5. ii FOREWORD The Office of the Federal Coordinator for Meteorological Services and Supporting Research (OFCM) works with Federal agency stakeholders to plan hurricane observing and reconnaissance in preparation for each hurricane season. -
Pacific Islands Meteorological Services in Action
PacifiC iSlAndS Meteorological ServiCeS in ACtion A Compendium of Climate Services Case Studies Compendium prepared by SPREP in partnership with WMO and Environment and Climate Change Canada Compiled by Christina Leala-Gale, FINPAC Project Manager, SPREP Reviewed by: Dr Netatua Pelesikoti, Director, Climate Change Division, SPREP and PICS Panel Members: Ofa Faʹanunu (Tonga Meteorological Service) Simon McGree (BoM) Neil Plummer (BoM) Molly Powers-Tora (BoM) Grant Smith (BoM) Dean Solofa (SPC) Andrew Tait (NIWA) SPREP Climate Change Division staff Secretariat of the Pacific Regional Environment Programme (SPREP) PO Box 240 Apia, Samoa [email protected] www.sprep.org Our vision: A resilient Pacific environment sustaining our livelihoods and natural heritage in harmony with our cultures. Copyright © Secretariat of the Pacific Regional Environment Programme (SPREP), 2016. Reproduction for educational or other non-commercial purposes is authorised without prior written permission from the copyright holder provided that the source is fully acknowledged. Reproduction of this publication for resale or other commercial purposes is prohibited without prior written consent of the copyright owner. Cover photo: Mr Posa Skelton SPREP Library Cataloguing-in-Publication Data Pacific Islands Meteorological Services in Action : a compendium of climate services case studies / compiled by Christina Leala-Gale. Apia, Samoa : SPREP, 2016. 100 p. 29 cm. ISBN: 978-982-04-0633-9 (print) 978-982-04-0634-6 (e-copy) 1. Meteorological services – Oceania. 2. Meteorological services – Case studies – Oceania. 3. Climatology – Case studies – Oceania. I. Leala-Gale, Christina. II. Pacific Regional Environment Programme (SPREP). III. Title. 551.510996 The Pacific Islands Climate Services (PICS) Panel endorsed by the Pacific Meteorological Council in July 2013 and established in March 2014 is a technical expert group for Climate Services. -
2019 National Hurricane Operations Plan
U.S. DEPARTMENT OF COMMERCE/ National Oceanic and Atmospheric Administration OFFICE OF THE FEDERAL COORDINATOR FOR METEOROLOGICAL SERVICES AND SUPPORTING RESEARCH National Hurricane Operations Plan FCM-P12-2019 Washington, DC May 2019 Hurricane Florence, courtesy of NOAA. FEDERAL COORDINATOR FOR METEOROLOGICAL SERVICES AND SUPPORTING RESEARCH 1325 East-West Highway, SSMC2 Silver Spring, Maryland 20910 301-628-0112 NATIONAL HURRICANE OPERATIONS PLAN FCM-P12-2019 Washington, D.C. May 2019 CHANGE AND REVIEW LOG Use this page to record changes and notices of reviews. Change Page Numbers Date Posted Initials Number (mm/dd/yyyy) 1. 2. 3. 4. 5. Changes are indicated by a vertical line in the margin next to the change or by shading and strikeouts. No. Review Date Comments Initials (mm/dd/yyyy) 1. 2. 3. 4. 5. iv FOREWORD The Office of the Federal Coordinator for Meteorological Services and Supporting Research (OFCM) works with Federal agency stakeholders to plan hurricane observing and reconnaissance in preparation for each hurricane season. OFCM’s Working Group for Tropical Cyclone Operations and Research (WG/TCOR) manages the process of publishing an annual update to the National Hurricane Operations Plan (NHOP), which documents the agreed-upon interagency plans. The NHOP focuses heavily on the planning, execution, and use of aerial reconnaissance conducted by the Air Force Reserve Command’s 53rd Weather Reconnaissance Squadron (WRS) and NOAA’s Aircraft Operations Center (AOC); addresses meteorological satellite, weather radar, and ocean observing; -
Effects of the Coriolis Force on Intensity of Hurricane PALI in Ensemble Experiments
AAS06-P07 JpGU-AGU Joint Meeting 2017 Effects of the Coriolis Force on Intensity of Hurricane PALI in Ensemble Experiments *Hiroaki Yoshioka1, Takeshi Enomoto2 1. Graduate School of Science, Kyoto University, 2. Disaster Prevention Research Institute, Kyoto University Tropical cyclones (TCs) observations are unusual in the equatorial region from 5°N to 5 °S. Therefore, previous studies studied the effects of the Coriolis force on TC in ideal experiments. These studies, however, have not shown the mechanisms for the TC intensification for realistic settings. Thus, the aim of this study is to clarify the mechanisms of the intensification of Hurricane PALI and the effects of the Coriolis force using a regional atmospheric model. PALI occurred at 4.4°N where is nearly 200 km southeast of Oahu island, USA, on 18Z 7 January 2016 and developed to 43.7 m×s-1 at the mature stage (Category-2) on 18Z 12. We conducted ensemble downscale experiments to deal with initial uncertainty. The non-hydrostatic mesoscale numerical model, WRF, with horizontal resolution of 10 km is used in the present study. To identify the effects of the Coriolis force, sensitivity experiments with Coriolis parameters are conducted. The sensitivity experiments were conducted by inputting the Coriolis forces relatively north to 10° every 1° from the calculation domain. The initial states were derived from 11 ensemble members of NOAA’s 2nd-generation global ensemble reforecast dataset. NCEP FNL (Final) Operational Global Analysis data is used as common soil data for all ensemble members and sea surface temperature fixed at the initial time. Among other settings, we used the Kain-Fritsch scheme for cumulus convection parameterization.