Quantum Gates and Memory Using Microwave-Dressed States
Total Page:16
File Type:pdf, Size:1020Kb
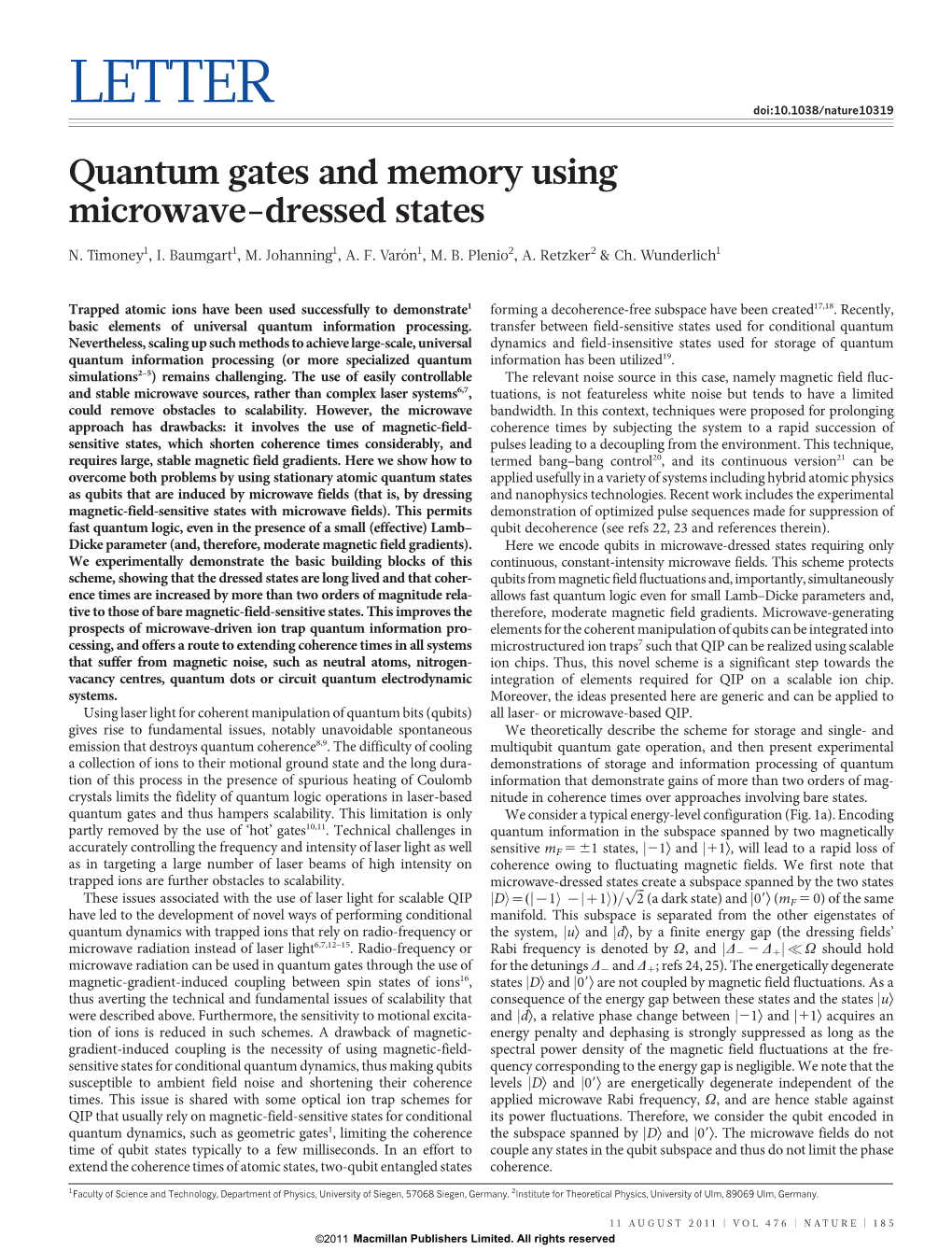
Load more
Recommended publications
-
Cond-Mat.Supr-Con] 1 Sep 2004 Hnn B Lcutoso H W Ee Ytm Nteoxi the in Systems Level Two 1 the of Fluctuations (B) De Phonon
Decoherence of a Josephson qubit due to coupling to two level systems Li-Chung Ku and C. C. Yu Department of Physics, University of California, Irvine, California 92697, U.S.A. (Dated: October 30, 2018) Abstract Noise and decoherence are major obstacles to the implementation of Josephson junction qubits in quantum computing. Recent experiments suggest that two level systems (TLS) in the oxide tunnel barrier are a source of decoherence. We explore two decoherence mechanisms in which these two level systems lead to the decay of Rabi oscillations that result when Josephson junction qubits are subjected to strong microwave driving. (A) We consider a Josephson qubit coupled resonantly to a two level system, i.e., the qubit and TLS have equal energy splittings. As a result of this resonant interaction, the occupation probability of the excited state of the qubit exhibits beating. Decoherence of the qubit results when the two level system decays from its excited state by emitting a phonon. (B) Fluctuations of the two level systems in the oxide barrier produce fluctuations and 1/f noise in the Josephson junction critical current Io. This in turn leads to fluctuations in the qubit energy splitting that degrades the qubit coherence. We compare our results with experiments on Josephson junction phase qubits. PACS numbers: 03.65.Yz, 03.67.Lx, 85.25.Cp arXiv:cond-mat/0409006v1 [cond-mat.supr-con] 1 Sep 2004 1 I. INTRODUCTION The Josephson junction qubit is a leading candidate as a basic component of a quantum computer. A significant advantage of this approach is scalability, as these qubits may be readily fabricated in large numbers using integrated-circuit technology. -
Pulsed Nuclear Magnetic Resonance
Pulsed Nuclear Magnetic Resonance Experiment NMR University of Florida | Department of Physics PHY4803L | Advanced Physics Laboratory References Theory C. P. Schlicter, Principles of Magnetic Res- Recall that the hydrogen nucleus consists of a onance, (Springer, Berlin, 2nd ed. 1978, single proton and no neutrons. The precession 3rd ed. 1990) of a bare proton in a magnetic field is a sim- ple consequence of the proton's intrinsic angu- E. Fukushima, S. B. W. Roeder, Experi- lar momentum and associated magnetic dipole mental Pulse NMR: A nuts and bolts ap- moment. A classical analog would be a gyro- proach, (Perseus Books, 1981) scope having a bar magnet along its rotational M. Sargent, M.O. Scully, W. Lamb, Laser axis. Having a magnetic moment, the proton Physics, (Addison Wesley, 1974). experiences a torque in a static magnetic field. Having angular momentum, it responds to the A. C. Melissinos, Experiments in Modern torque by precessing about the field direction. Physics, This behavior is called Larmor precession. The model of a proton as a spinning positive Introduction charge predicts a proton magnetic dipole mo- ment µ that is aligned with and proportional To observe nuclear magnetic resonance, the to its spin angular momentum s sample nuclei are first aligned in a strong mag- netic field. In this experiment, you will learn µ = γs (1) the techniques used in a pulsed nuclear mag- netic resonance apparatus (a) to perturb the where γ, called the gyromagnetic ratio, would nuclei out of alignment with the field and (b) depend on how the mass and charge is dis- to measure the small return signal as the mis- tributed within the proton. -
Semiclassical Approach to Atomic Decoherence by Gravitational Waves
Semiclassical approach to atomic decoherence by gravitational waves Diego A. Qui˜nones, Benjamin Varcoe August 2, 2018 Abstract A new heuristic model of interaction of an atomic system with a gravitational wave is proposed. In it, the gravitational wave alters the local electromagnetic field of the atomic nucleus, as perceived by the electron, changing the state of the system. The spectral decomposition of the wave function is calculated, from which the energy is obtained. The results suggest a shift in the difference of the atomic energy lev- els, which will induce a small detuning to a resonant transition. The detuning increases with the quantum numbers of the levels, making the effect more prominent for Rydberg states. We performed calcu- lations on the Rabi oscillations of atomic transitions, estimating how they would vary as a result of the proposed effect. 1 Introduction In general relativity, gravity is the curvature of the space-time continuum produced by the mass of objects [41]. Similar to how accelerating electrical charges produce electromagnetic waves, accelerating masses will produce rip- ples in the fabric of space-time [16, 17], which are called gravitational waves (GWs). They are predicted to exist in a very wide range of frequencies, de- pending on the source that generate them [2, 11, 14, 27, 29, 30, 37, 39, 40, 44], but even the most energetic ones (like the ones produced by rotating neutron stars [3]) will only produce a very small distortion of space, making them arXiv:1706.01287v3 [quant-ph] 1 Dec 2017 very hard to detect. Although GWs have been previously observed indirectly [43], it was only re- cently that a direct detection was performed by analyzing the signal of very big interferometers [18–20]. -
Charge Qubit Coupled to Quantum Telegraph Noise
Charge Qubit Coupled to Quantum Telegraph Noise Florian Schröder München 2012 Charge Qubit Coupled to Quantum Telegraph Noise Florian Schröder Bachelorarbeit an der Fakultät für Physik der Ludwig–Maximilians–Universität München vorgelegt von Florian Schröder aus München München, den 20. Juli 2012 Gutachter: Prof. Dr. Jan von Delft Contents Abstract vii 1 Introduction 1 1.1 Time Evolution of Closed Quantum Systems . .... 2 1.1.1 SchrödingerPicture........................... 2 1.1.2 HeisenbergPicture ........................... 3 1.1.3 InteractionPicture ........................... 3 1.2 Description of the System of Qubit and Bath . ..... 4 2 Quantum Telegraph Noise Model 7 2.1 Hamiltonian................................... 7 2.2 TheMasterEquation.............................. 7 2.3 DMRGandModelParameters. 11 2.3.1 D, ∆, γ and ǫd ............................. 11 2.3.2 DiscretizationandBathLength . 11 3 The Periodically Driven Qubit 15 3.1 TheFullModel ................................. 15 3.2 RabiOscillations ................................ 15 3.2.1 Pulses .................................. 17 3.3 Bloch-SiegertShift .............................. 18 3.3.1 Finding the Bloch-Siegert Shift . .. 18 3.4 DrivenQubitCoupledtoQTN ........................ 21 3.5 Simulations of Spin Echo and Bang-Bang with Ideal π-Pulses. 23 4 Conclusion and Outlook 27 A Derivations 29 A.1 MasterEquation ................................ 29 A.1.1 ProjectionOperatorMethod. 29 A.1.2 Interaction Picture Hamiltonian . ... 31 A.1.3 BathCorrelationFunctions . 33 A.2 DiscreteFourierTransformation . ..... 36 vi Contents A.3 SolutionoftheRabiProblem . 39 A.3.1 Rotating-Wave-Approximation . .. 39 A.3.2 Bloch-SiegertShift ........................... 40 A.4 Jaynes-Cummings-Hamiltonian . ... 41 Bibliography 47 Acknowledgements 48 Abstract I simulate the time evolution of a qubit which is exposed to quantum telegraph noise (QTN) with the time-dependent density matrix renormalization group (t-DMRG). -
Vortices in a Trapped Dilute Bose–Einstein Condensate
INSTITUTE OF PHYSICS PUBLISHING JOURNAL OF PHYSICS: CONDENSED MATTER J. Phys.: Condens. Matter 13 (2001) R135–R194 www.iop.org/Journals/cm PII: S0953-8984(01)08644-1 TOPICAL REVIEW Vortices in a trapped dilute Bose–Einstein condensate Alexander L Fetter and Anatoly A Svidzinsky Department of Physics, Stanford University, Stanford, CA 94305-4060, USA Received 29 November 2000, in final form 2 February 2001 Abstract We review the theory of vortices in trapped dilute Bose–Einstein condensates and compare theoretical predictions with existing experiments. Mean-field theory based on the time-dependent Gross–Pitaevskii equation describes the main features of the vortex states, and its predictions agree well with available experimental results. We discuss various properties of a single vortex, including its structure, energy, dynamics, normal modes, and stability, as well as vortex arrays. When the nonuniform condensate contains a vortex, the excitation spectrum includes unstable (‘anomalous’) mode(s) with negative frequency. Trap rotation shifts the normal-mode frequencies and can stabilize the vortex. We consider the effect of thermal quasiparticles on vortex normal modes as well as possible mechanisms for vortex dissipation. Vortex states in mixtures and spinor condensates are also discussed. Contents 1. Introduction 2. The time-dependent Gross–Pitaevskii equation 2.1. Unbounded condensate 2.2. Quantum-hydrodynamic description of the condensate 2.3. Vortex dynamics in two dimensions 2.4. Trapped condensate 3. Static vortex states 3.1. Structure of a single trapped vortex 3.2. Thermodynamic critical angular velocity for vortex stability 3.3. Experimental creation of a single vortex 3.4. Vortex arrays 4. -
Switchable Resonant Hyperpolarization Transfer from Phosphorus-31 Nuclei to Silicon-29 Nuclei in Natural Silicon
Switchable resonant hyperpolarization transfer from phosphorus-31 nuclei to silicon-29 nuclei in natural silicon by Phillip Dluhy B.Sc., Loyola University Chicago, 2012 Thesis Submitted in Partial Fulfillment of the Requirements for the Degree of Master of Science in the Department of Physics Faculty of Science c Phillip Dluhy 2015 SIMON FRASER UNIVERSITY Summer 2015 All rights reserved. However, in accordance with the Copyright Act of Canada, this work may be reproduced without authorization under the conditions for “Fair Dealing.” Therefore, limited reproduction of this work for the purposes of private study, research, criticism, review and news reporting is likely to be in accordance with the law, particularly if cited appropriately. Approval Name: Phillip Dluhy Degree: Master of Science (Physics) Title: Switchable resonant hyperpolarization transfer from phosphorus-31 nuclei to silicon-29 nuclei in natural silicon Examining Committee: Chair: Dr. Malcolm Kennett Associate Professor Dr. Michael L. W. Thewalt Senior Supervisor Professor Dr. David Broun Supervisor Associate Professor Dr. Simon Watkins Internal Examiner Professor Department of Physics Date Defended: 01 May 2015 ii Abstract Silicon has been the backbone of the microelectronics industry for decades. As spin-based technologies continue their rapid development, silicon is emerging as a material of primary interest for a number of these applications. There are several techniques that currently 1 29 exist for polarizing the spin- 2 Si nuclei, which account for 4.7% of the isotopic makeup of natural silicon (the other two stable isotopes, 28Si and 30Si, have zero nuclear spin). Polar- ized 29Si nuclei may find use in quantum computing (QC) implementations and magnetic resonance (MR) imaging. -
Arxiv:1212.5864V2 [Quant-Ph]
Vacuum Rabi oscillation induced by virtual photons in the ultrastrong coupling regime C. K. Law Department of Physics and Institute of Theoretical Physics, The Chinese University of Hong Kong, Shatin, Hong Kong Special Administrative Region, People’s Republic of China We present an interaction scheme that exhibits a dynamical consequence of virtual photons carried by a vacuum-field dressed two-level atom in the ultrastrong coupling regime. We show that, with the aid of an external driving field, virtual photons provide a transition matrix element that enables the atom to evolve coherently and reversibly to an auxiliary level accompanied by the emission of a real photon. The process corresponds to a type of vacuum Rabi oscillation, and we show that the effective vacuum Rabi frequency is proportional to the amplitude of a single virtual photon in the ground state. Therefore the interaction scheme could serve as a probe of ground state structures in the ultrastrong coupling regime. PACS numbers: 42.50.Pq, 42.50.Ct, 42.50.Lc A single-mode electromagnetic field interacting with a f two-level atom has been a fundamental model in quan- ω tum optics capturing the physics of resonant light-matter p interaction. In particular, the Jaynes-Cummings (JC) model [1, 2], which describes the regime where the inter- e action energy ~λ is much smaller than the energy scale ω c of an atom ~ωA and a photon ~ωc, has tremendous ap- g plications in cavity QED [3, 4] and trapped ion systems [5]. Recently, there has been considerable research inter- FIG. 1: (Color online) Interaction scheme of a Ξ−type three- est in the ultrastrong coupling regime where λ becomes level atom in a cavity. -
Synthetic Spin–Orbit Coupling and Topological Polaritons in Janeys–Cummings Lattices
www.nature.com/npjqi ARTICLE OPEN Synthetic spin–orbit coupling and topological polaritons in Janeys–Cummings lattices Feng-Lei Gu 1, Jia Liu1, Feng Mei2,3, Suotang Jia2,3, Dan-Wei Zhang1 and Zheng-Yuan Xue1 The interaction between a photon and a qubit in the Janeys–Cummings (JC) model generates a kind of quasiparticle called polariton. While they are widely used in quantum optics, difficulties in engineering-controllable coupling of them severely limit their applications to simulate spinful quantum systems. Here we show that, in the superconducting quantum circuit context, polariton states in the single-excitation manifold of a JC lattice can be used to simulate a spin-1/2 system, based on which tunable synthetic spin–orbit coupling and novel topological polaritons can be generated and explored. The lattice is formed by a sequence of coupled transmission line resonators, each of which is connected to a transmon qubit. Synthetic spin–orbit coupling and the effective Zeeman field of the polariton can both be tuned by modulating the coupling strength between neighboring resonators, allowing for the realization of a large variety of polaritonic topological semimetal bands. Methods for detecting the polaritonic topological edge states and topological invariants are also proposed. Therefore, our work suggests that the JC lattice is a versatile platform for exploring spinful topological states of matter, which may inspire developments of topologically protected quantum optical and information-processing devices. npj Quantum Information (2019) 5:36 ; https://doi.org/10.1038/s41534-019-0148-9 INTRODUCTION lattices.31–34 However, the limited trapping time and the site- The Janeys–Cummings (JC) model proposed in 19631 is a seminal addressing difficulty increase the experimental complexity, i.e., it is theoretical model treating light–matter interaction with full generally difficult to have modulable coupling between two quantum theory, i.e., the interaction of a quantized electromagnetic neighboring sites in an optical lattice. -
Measurement-Driven Dynamics for a Coherently-Excited Atom
October 26, 2012 9:15 Journal of Modern Optics (4)*Measurement-driven*dynamics*for*a*coherently- excited*atom Journal of Modern Optics Vol. 00, No. 00, 00 Month 200x, 1{20 Measurement-driven dynamics for a coherently-excited atom Andrew J.T. Colina∗ Stephen M. Barnetta and John Jeffersa aDepartment of Physics, University of Strathclyde, John Anderson Building, Glasgow G4 0NG, UK; (v1.0 released October 2012) The phenomenon of telegraphing in a measurement-driven two-level atom was noted in [1]. Here we introduce two quantitative measures of telegraphing: one based on the accumulated measurement record and one on the evolution of the quantum state. We use these to analyse the dynamics of the atom over a wide range of parameters. We find, in particular, that the measures provide broadly similar statistics when the measurements are frequent, but differ widely when measurements are sparse. This is in line with intuition, and demonstrates the utility of both measures. Keywords: Master equations; Lindblad form; Markov processes; Telegraphing; Rabi cycle; Two-state atoms; Imperfect measurements ∗Corresponding author. Email: [email protected] ISSN: 0950-0340 print/ISSN 1362-3044 online c 200x Taylor & Francis DOI: 10.1080/0950034YYxxxxxxxx http://www.informaworld.com October 26, 2012 9:15 Journal of Modern Optics (4)*Measurement-driven*dynamics*for*a*coherently- excited*atom 2 1. Introduction The idea of random telegraph dynamics for a single quantum system started with the proposal by Dehmelt [2] to detect a weak transition by monitoring the fluorescence on a strong one, as depicted in Figure 1 . The fluorescence observed on the strong transition is interrupted by periods of darkness during which, we can infer, an absorption has occurred on the weak transition [3, 4]. -
Quantum Trajectory Analysis of a Thresholdlike Transition in the Microlaser
PHYSICAL REVIEW A VOLUME 55, NUMBER 6 JUNE 1997 Quantum trajectory analysis of a thresholdlike transition in the microlaser Changhuei Yang and Kyungwon An George R. Harrison Laboratory, Massachusetts Institute of Technology, Cambridge, Massachusetts 02139 ~Received 29 July 1996! In a recent microlaser experiment @K. An et al., Phys. Rev. Lett. 73, 3375 ~1994!#, a thresholdlike transition of intracavity mean photon number as a function of intracavity mean atom number has been observed. In this paper the behavior is explored with quantum trajectory simulations. It is shown that the transition is caused by enhanced atom-cavity Rabi interaction due to the increase of the intracavity photon number as the intracavity atom number is increased. The transition is further accentuated by the position-dependent variation of the coupling constant in the Fabry-Pe´rot cavity. In addition, it is demonstrated that multiatom collective effects are negligible in the microlaser under consideration, in which atoms are injected into the cavity at random times and the product of the coupling constant and atom-cavity interaction time is much less than p. In this case the analytic theory of the one-atom micromaser @P. Filipowicz et al., Phys. Rev. A 34, 3077 ~1986!# can be extrapolated into the multiatom region, assuming uniform atom-cavity coupling throughout the cavity and monovelocity atomic injection. Finally, simulations are performed which account for spatial variation of coupling constant, velocity distribution of injected atoms, and spontaneous atomic decay in the actual experi- ment. The results are in good agreement with experiment. @S1050-2947~97!03506-3# PACS number~s!: 42.50.2p, 42.55.2f, 32.80.2t I. -
Breaking the Trade-Off Between Fast Control and Long Lifetime of A
Breaking the trade-off between fast control and long lifetime of a superconducting qubit S. Kono1, K. Koshino2, D. Lachance-Quirion3;4, A. F. van Loo1, Y. Tabuchi3, A. Noguchi5;6, and Y. Nakamura1;3 1Center for Emergent Matter Science (CEMS), RIKEN, Wako, Saitama 351-0198, Japan 2College of Liberal Arts and Sciences, Tokyo Medical and Dental University, Ichikawa, Chiba 272-0827, Japan 3Research Center for Advanced Science and Technology (RCAST), The University of Tokyo, Meguro-ku, Tokyo 153-8904, Japan 4Institut quantique and Dpartement de physique, Universit de Sherbrooke, Sherbrooke, Qubec, J1K 2R1, Canada 5Komaba Institute for Science (KIS), The University of Tokyo, Meguro-ku, Tokyo, 153-8902, Japan and 6PRESTO, Japan Science and Technology Agency, Kawaguchi-shi, Saitama 332-0012, Japan (Dated: March 10, 2020) The rapid development in designs and fabrication techniques of superconducting qubits has made coherence times of qubits longer. In the near future, however, the radiative decay of a qubit into its control line will be a fundamental limitation, imposing a trade-off between fast control and long lifetime of the qubit. In this work, we successfully break this trade-off by strongly coupling another superconducting qubit along the control line. This second qubit, which we call a Josephson quantum filter (JQF), prevents the qubit from emitting microwave photons and thus suppresses its relaxation, while faithfully transmitting large-amplitude control microwave pulses due to the saturation of the quantum filter, enabling fast qubit control. We observe an improvement of the qubit relaxation time without a reduction of the Rabi frequency. This device could potentially help in the realization of a large-scale superconducting quantum information processor in terms of the heating of the qubit environment and the crosstalk between qubits. -
Arxiv:2105.05188V1
Cavity driven Rabi oscillations between Rydberg states of atoms trapped on a superconducting atom chip 1, 1, 1 1 1 1 Manuel Kaiser, ∗ Conny Glaser, † Li Yuan Ley, Jens Grimmel, Helge Hattermann, Daniel Bothner, 1 1 1, 2 1, 1, Dieter Koelle, Reinhold Kleiner, David Petrosyan, Andreas G¨unther, ‡ and J´ozsef Fort´agh § 1Center for Quantum Science, Physikalisches Institut, Eberhard Karls Universit¨at T¨ubingen, Auf der Morgenstelle 14, D-72076 T¨ubingen, Germany 2Institute of Electronic Structure and Laser, FORTH, GR-70013 Heraklion, Crete, Greece (Dated: May 12, 2021) Hybrid quantum systems involving cold atoms and microwave resonators can enable cavity- mediated infinite-range interactions between atomic spin systems and realize atomic quantum mem- ories and transducers for microwave to optical conversion. To achieve strong coupling of atoms to on-chip microwave resonators, it was suggested to use atomic Rydberg states with strong electric dipole transitions. Here we report on the realization of coherent coupling of a Rydberg transition of ultracold atoms trapped on an integrated superconducting atom chip to the microwave field of an on-chip coplanar waveguide resonator. We observe and characterize the cavity driven Rabi oscil- lations between a pair of Rydberg states of atoms in an inhomogeneous electric field near the chip surface. Our studies demonstrate the feasibility, but also reveal the challenges, of coherent state manipulation of Rydberg atoms interacting with superconducting circuits. INTRODUCTION one of the most accurately controlled quantum optical systems [24]. Long-lived coherence of Rydberg state Developing various hardware components for quantum superpositions of atoms above a superconducting chip information processing, storage and communication, as have been demonstrated [25].