NCAR/TN-237+IA Instructor's Handbook On
Total Page:16
File Type:pdf, Size:1020Kb
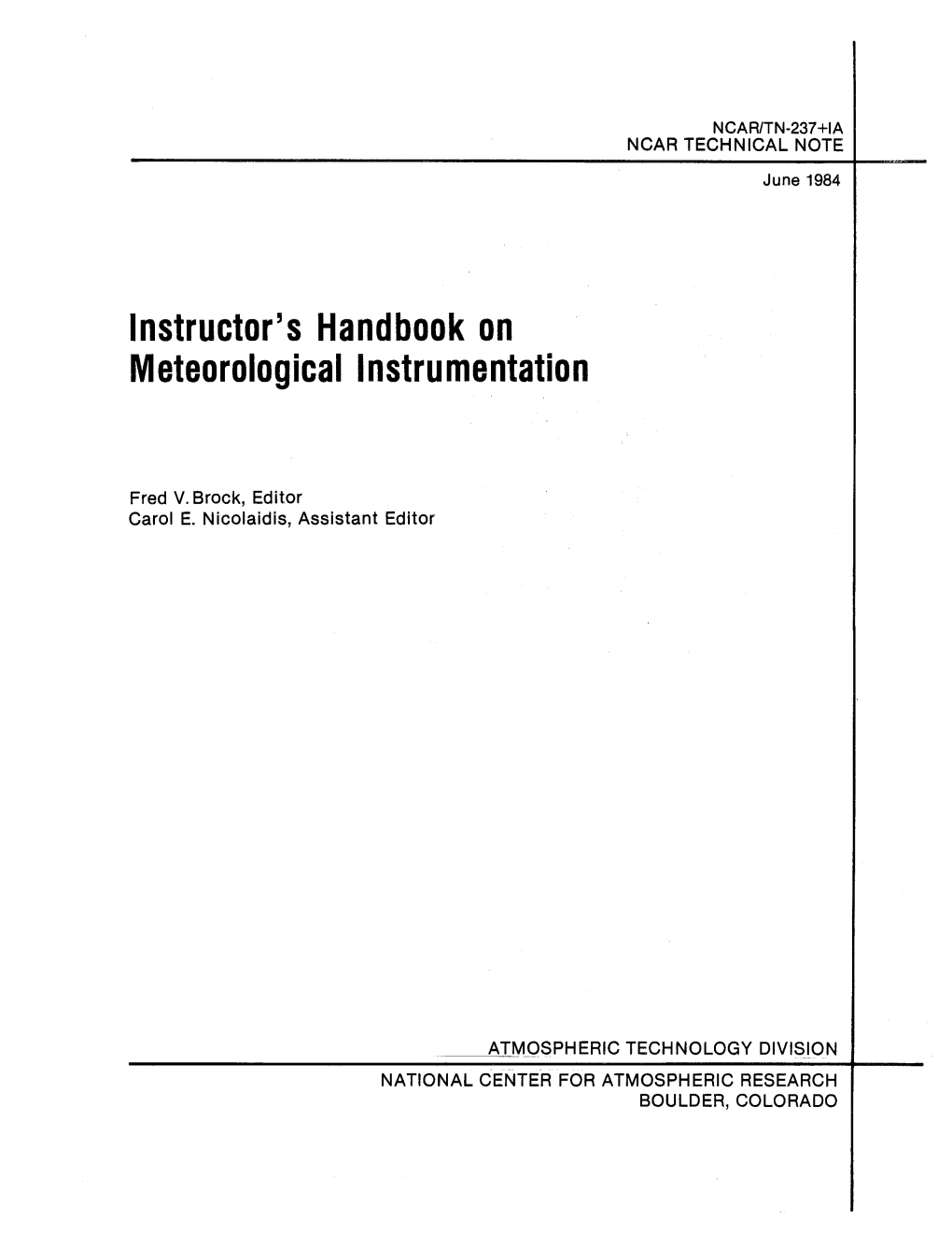
Load more
Recommended publications
-
Climate Data Sources in Connecticut Patricia A
University of Connecticut OpenCommons@UConn College of Agriculture, Health and Natural Storrs Agricultural Experiment Station Resources 1-1982 Climate Data Sources in Connecticut Patricia A. Palley University of Connecticut - Storrs David R. Miller University of Connecticut - Storrs Follow this and additional works at: https://opencommons.uconn.edu/saes Part of the Climate Commons, Environmental Monitoring Commons, and the Meteorology Commons Recommended Citation Palley, Patricia A. and Miller, David R., "Climate Data Sources in Connecticut" (1982). Storrs Agricultural Experiment Station. 80. https://opencommons.uconn.edu/saes/80 Storrs Agricultural Experiment Station Bulletin 461 Climate Data Sources in Connecticut By Patricia A Palley, Assistant State Climatologist and David R. Miller, Associate Professor of Natural Resources JAN 1982 STORRS AGRICULTURAL EXPERI MENT STATION COLLEGE OF AGRICULTURE AND NATURAL RESOURCES THE UNIVERSITY OF CONNECTICUT, STORRS. CT 06268 TABLE OF CONTENTS Int roduction . 1 Types of Weather Stations 2 Parameters Measur ed 3 Summary of Climate Observations in Connecticu t 5 How to Use the Maps and Site Reports • • • • • 7 Table I Record Lengths, by parameter, of all weather stat ions i n Conn., state summary 8 Table II Record Lengths , by parame ter, of all weather stations in Conn . , by county . 9 Table III Record Lengths, by paramet er, of Nationa l Wea ther Service operat ed and coope rative stations in Conn., by county . • . 10 Table IV Re cord Lengths , by par ameter , of pr ivate data collect ors i n Conn ., by county . • . 11 Figure I Distribution of stations t hat measure rainfall . 12 Figur e II Distribution of stations t hat meas ure s nowf all . -
Glossary Terms
Glossary Terms € 1584 5W6 5501 a 7181, 12203 5’UTR 8126 a-g Transformation 6938 6Q1 5500 r 7181 6W1 5501 b 7181 a 12202 b-b Transformation 6938 A 12202 d 7181 AAV 10815 Z 1584 Abandoned mines 6646 c 5499 Abiotic factor 148 f 5499 Abiotic 10139, 11375 f,b 5499 Abiotic stress 1, 10732 f,i, 5499 Ablation 2761 m 5499 ABR 1145 th 5499 Abscisic acid 9145 th,Carnot 5499 Absolute humidity 893 th,Otto 5499 Absorbed dose 3022, 4905, 8387, 8448, 8559, 11026 v 5499 Absorber 2349 Ф 12203 Absorber tube 9562 g 5499 Absorption, a(l) 8952 gb 5499 Absorption coefficient 309 abs lmax 5174 Absorption 309, 4774, 10139, 12293 em lmax 5174 Absorptivity or absorptance (a) 9449 μ1, First molecular weight moment 4617 Abstract community 3278 o 12203 Abuse 6098 ’ 5500 AC motor 11523 F 5174 AC 9432 Fem 5174 ACC 6449, 6951 r 12203 Acceleration method 9851 ra,i 5500 Acceptable limit 3515 s 12203 Access time 1854 t 5500 Accessible ecosystem 10796 y 12203 Accident 3515 1Q2 5500 Acclimation 3253, 7229 1W2 5501 Acclimatization 10732 2W3 5501 Accretion 2761 3 Phase boundary 8328 Accumulation 2761 3D Pose estimation 10590 Acetosyringone 2583 3Dpol 8126 Acid deposition 167 3W4 5501 Acid drainage 6665 3’UTR 8126 Acid neutralizing capacity (ANC) 167 4W5 5501 Acid (rock or mine) drainage 6646 12316 Glossary Terms Acidity constant 11912 Adverse effect 3620 Acidophile 6646 Adverse health effect 206 Acoustic power level (LW) 12275 AEM 372 ACPE 8123 AER 1426, 8112 Acquired immunodeficiency syndrome (AIDS) 4997, Aerobic 10139 11129 Aerodynamic diameter 167, 206 ACS 4957 Aerodynamic -
Radiation and Climate
m ^ <(. -Zc'.^.^Z l INTERNATIONAL COUNCIL OF WORLD METEOROLOGICAL SCIENTIFIC UNIONS ORGANIZATION RADIATION AND CLIMATE SECOND WORKSHOP ON IMPLEMENTATION OF THE BASELINE SURFACE RADIATION NETWORK (Davos, Switzerland, 6-9 August 1991) NOVEMBER 1991 WCRP-64 WMO/TD-No. 453 ) i t 'I The World Climate Programme launched by the World Meteorological Organization (WMO) includes four components: The World Climate Data and Monitoring Programme The World Climate Applications and Services Programme The World Climate Impact Assessment and Response Strategies Programme The World Climate Research Programme The World Climate Research Programme is jointly sponsored by the WMO and the International Council of Scientific Unions. I NOTE The designations employed and the presentation of material in this publication do not imply the expression of any opinion whatsoever on the part of the Secretariat of the World Meteorological Organizaüon concerning the legal status of any country, territory, city or area, or of its authoriues, or concerning the delimitation of its frontiers or boundaries. This report has been produced without editorial revision by the WMO Secretariat. It is not an official publication and its distribution in this form does not imply endorsement by the Organization of the ideas expressed. R. t; TABLE OF CONTENTS Page No. l. OBJECTIVES OF WORKSHOP l 2. REPORTS ON INSTRUMENTATION AND MEASUREMENT UNCERTAINTY STUDIES 2 3. RECOMMENDATIONS FOR DETERMINATION OF BASIC BSRN PARAMETERS AND BSKN OPERATIONAL PROCEDURES 8 '9^ 3.l Instruments and methods 8 •i:'K.'"- 3.2 Calibration procedures 11 3.3 Data acquisition 12 3.4 Measurement uncertainty techniques 13 3.5 Preparation of operations manual for the BSRN 13 4. -
Lidar Supplied to Brazil Calibrating the Italian Air Force Brewer Network
News Letter 8 Lidar supplied to Brazil Calibrating the Italian Air Force Brewer Network LAS helps to improve Water Management in Australia A Special Sun Tracker Application in the Arctic Exciting Things coming Are you the next Kipp & Zonen award winner? up this Spring Every year, during the EMS Meeting, we grant the award to an outstanding research paper on Boundary Layer Following the article on the development of the new Meteorology by a young aspiring scientist. Go to our CNR 4 net radiometer in the previous newsletter, I am newspage on www.kippzonen.com for more information. happy to confirm that it is now available for ordering. The interest from customers is very promising and we have high expectations. Read all about the light weight, optional integrated ventilation, standard PT-100 and Content April 2009 thermistor temperature sensors, and other innovative features, on page 3 or the CNR 4 product page at www.kippzonen.com. P2: Ben’s Column Exciting Things coming up this Spring You will have seen our completely new website last year and we are continuing to improve it and add extra functionalities. For P3: News update example, we recently launched a new tool to speed up product CNR 4 Net Radiometer inquiries and quotation requests. Future possibilities include AMS 2009 in Phoenix sub-sites focussed on specific markets and language options. Lidar supplied to Brazil Behind the scenes, we have also made some changes and you P4: Calibrating the Italian Air Force Brewer Network may have noticed the benefits already. We have completed the integration of a new ERP system that allows us to plan production P5: Kipp & Zonen LAS helps to improve and manage logistics better. -
Measuring Routines of Ice Accretion for Wind Turbine Applications
Measuring routines of ice accretion for Wind Turbine applications The correlation of production losses and detection of ice Viktor Carlsson Viktor Carlsson Ht 2009 Master thesis, 30 hp Master of Science programme in Engineering Physics, 180 hp Master thesis in engineering physics 2010-11-18 Abstract Wind power will play a major role in the future energy system in Sweden. Most of the major wind parks are planned to be built in sites where the cold climate and atmospheric icing can cause serious problems. This underlines the importance of addressing these issues. The major cause of these problems is in-cloud icing of the rotor blades due to super cooled liquid droplets of clouds. The droplets freeze upon impact with the rotor blade and form hard rime ice. This rime ice causes disruption in the aerodynamics that leads to production losses, extra loads on the rotor blades and when the ice is shed it poses a safety risk to people in the near environment. This master thesis focuses on how to measure the accretion of ice and the correlation between measured ice and production losses of two wind parks in northern Sweden. The results show a good correlation between the ice accretion on a stationary sensor and the production loss from a wind turbine. In most icing events the icing of the sensor and large production losses from the wind turbine correlated clearly. Attempts to quantify the production losses at a certain ice rate measured with the stationary sensors was done, however no clear results was produced. The reason for this is that the wind turbines often stop completely during an icing event and that the time series analyzed was too short to be able to quantify the losses at certain wind speed and ice rates. -
Best Practices Handbook for the Collection and Use of Solar Resource Data for Solar Energy Applications: Second Edition
Best Practices Handbook for the Collection and Use of Solar Resource Data for Solar Energy Applications: Second Edition Edited by Manajit Sengupta,1 Aron Habte,1 Christian Gueymard,2 Stefan Wilbert,3 Dave Renné,4 and Thomas Stoffel5 1 National Renewable Energy Laboratory 2 Solar Consulting Services 3 German Aerospace Center (DLR) 4 Dave Renné Renewables, LLC 5 Solar Resource Solutions, LLC This update was prepared in collaboration with the International Energy Agency Solar Heating and Cooling Programme: Task 46 NREL is a national laboratory of the U.S. Department of Energy Office of Energy Efficiency & Renewable Energy Operated by the Alliance for Sustainable Energy, LLC This report is available at no cost from the National Renewable Energy Laboratory (NREL) at www.nrel.gov/publications. Technical Report NREL/TP-5D00-68886 December 2017 Contract No. DE-AC36-08GO28308 Best Practices Handbook for the Collection and Use of Solar Resource Data for Solar Energy Applications: Second Edition Edited by Manajit Sengupta,1 Aron Habte,1 Christian Gueymard,2 Stefan Wilbert,3 Dave Renné,4 and Thomas Stoffel5 1 National Renewable Energy Laboratory 2 Solar Consulting Services 3 German Aerospace Center (DLR) 4 Dave Renné Renewables, LLC 5 Solar Resource Solutions, LLC Prepared under Task No. SETP.10304.28.01.10 NREL is a national laboratory of the U.S. Department of Energy Office of Energy Efficiency & Renewable Energy Operated by the Alliance for Sustainable Energy, LLC This report is available at no cost from the National Renewable Energy Laboratory (NREL) at www.nrel.gov/publications. National Renewable Energy Laboratory Technical Report 15013 Denver West Parkway NREL/TP-5D00-68886 Golden, CO 80401 December 2017 303-275-3000 • www.nrel.gov Contract No. -
Weather Station XET C3VP
GPM Ground Validation Environment Canada (EC) Weather Station XET C3VP Introduction The GPM Ground Validation Environment Canada (EC) Weather Station XET C3VP dataset consists of surface meteorological data collected at the Environment Canada (EC) XET station at the Centre for Atmospheric Research Experiments (CARE) during the Canadian CloudSat/CALIPSO Validation Project (C3VP) field campaign. The campaign took place in southern Canada in support of multiple science missions, including the NASA GPM mission, in order to improve the modeling and remote sensing of winter precipitation. The XET C3VP dataset file includes temperature, pressure, wind speed and direction, relative humidity, solar radiation, grass temperature, soil temperature, snow depth, sunshine, and precipitation measurements from October 4, 2006 through March 31, 2007 in ASCII-csv format. Citation Rodriguez, Peter. 2020. GPM Ground Validation Environment Canada (EC) Surface Meteorological Station C3VP [indicate subset used]. Dataset available online from the NASA Global Hydrology Resource Center DAAC, Huntsville, Alabama, U.S.A. doi: http://dx.doi.org/10.5067/GPMGV/C3VP/METSTATION/DATA201 Keywords: NASA, GHRC, EC, C3VP, CARE, XET, air temperature, air pressure, wind speed/direction, relative humidity, solar radiation, sunshine, soil temperature, grass temperature, snow depth, precipitation Campaign The Global Precipitation Measurement mission Ground Validation (GPM GV) campaign used a variety of methods for validation of GPM satellite constellation measurements prior to and after launch of the GPM Core Satellite, which launched on February 27, 2014. The instrument validation effort included numerous GPM-specific and joint agency/international external field campaigns, using state of the art cloud and precipitation observational infrastructure (polarimetric radars, profilers, rain gauges, and disdrometers). -
News and Notes
Bulletin American Meteorological Society Miller, L. Jay, 1972: Dual-Doppler radar observations of Snider, J. B., 1972: Ground-based sensing of temperature circulation in snow conditions. Proc. 15th Radar Meteor. profiles from angular and multi-spectral emission measure- Conf., Amer. Meteor. Soc. ments. J. Appl. Meteor11, 958-967. Owens, J. C., 1969: Optical Doppler measurement of micro- scale wind velocity. Proc. IEEE, 57, 530-536. Strauch, R. G., V. E. Derr, and R. E. Cupp, 1971: Atmo- Richter, J. H., 1969: High resolution tropospheric radar spheric temperature measurement using Raman lidar. sounding. Radio Sci., 4, 1261-1268. Appl Opt., 10, 2665-1669. Salzman, J. A., W. J. Masica, and T. A. Coney, 1971: Deter- V. E. Derr, and R. E. Cupp, 1972: Atmospheric water mination of gas temperatures from laser-Raman scattering. vapor meaesurement using Raman lidar. Remote Sensing NASA TN D-6336. of Environment (in press). Schotland, R. M., 1969: Some aspects of remote atmospheric sensing by laser radar. Rept. of Remote Atrnos. Probing Wyngaard, J. E., Y. Izumi, and S. A. Collins, Jr., 1971: Be- Panel, Committee on Atrnos. Sci., Nat'l Acad, of Sci-Natl havior of the refractive-index-structure parameter near the Res. Council, 2, 179-200. ground. J. Opt. Soc. Amer., 61, 1646-1650. news and notes Lightning suppression by seeding with two chaff dispensers and field mills to measure electric field strength. Flying below cloud level, the NOAA scientists During a six-week long experiment, scientists from the waited until their instruments registered a field greater than National Oceanic and Atmospheric Administration attempted 30,000 volts per meter, a magnitude at which corona dis- to suppress lightning by seeding thunderstorms with fine charge will occur, When this happens the chaff dispensers aluminized fibers over a 200 mis area of northeastern Colo- are activated and the threadlike fibers are carried into the rado. -
Research Areas in Space
RESPOND & AI Capacity Building Programme Office ISRO HQ, Bengaluru RESPOND & AI Capacity Building Programme Office ISRO HQ, Bengaluru RESEARCH AREAS IN SPACE A Document for Preparing Research Project Proposals RESPOND & AI Capacity Building Programme Office ISRO HQ, Bengaluru January 2021 Technical Guidance Dr. M A Paul, Associate Director, RESPOND & AI, ISRO HQ Technical Support and Compilation Smt Nirupama Tiwari, Sci/Engr SE, CBPO, ISRO HQ Shri K Mahesh, Sr. Asst, CBPO, ISRO HQ Technical Guidance Dr. M A Paul, Associate Director, RESPOND & AI, ISRO HQ For any queries please contact Director, Capacity Building Programme Office (CBPO) Technical Support and Compilation Indian Space Research Organisation HQ Smt Nirupama Tiwari, Sci/Engr SE, CBPO, ISRO HQ Department of Space Shri K Mahesh, Sr. Asst, CBPO, ISRO HQ Government of India Antariksh Bhavan New BEL Road For any queries please contact Bangalore 560094 E-mail: [email protected] Director, Capacity Building Programme Office (CBPO) Indian Space Research Organisation HQ Department of Space Associate Director, RESPOND & Academic Interface Government of India Indian Space Research Organisation HQ Antariksh Bhavan Department of Space New BEL Road Government of India Bangalore 560094 Antariksh Bhavan E-mail: [email protected] New BEL Road Bangalore 560094 Associate Director, RESPOND & Academic Interface E-mail: [email protected] Indian Space Research Organisation HQ Department of Space Government of India Antariksh Bhavan New BEL Road Bangalore 560094 E-mail: [email protected] CONTENTS -
Remote and Autonomous Measurements of Precipitation for the Northwest Ross Ice Shelf, Antarctica Mark W
Discussions https://doi.org/10.5194/essd-2021-163 Earth System Preprint. Discussion started: 24 June 2021 Science c Author(s) 2021. CC BY 4.0 License. Open Access Open Data Remote and Autonomous Measurements of Precipitation for the Northwest Ross Ice Shelf, Antarctica Mark W. Seefeldt1, Taydra M. Low2, Scott D. Landolt3, Thomas H. Nylen4 1National Snow Ice and Data Center, University of Colorado Boulder, Boulder, CO, 80303, USA 5 2Department of Atmospheric and Oceanic Sciences, University of Colorado Boulder, Boulder, CO, 80303, USA 3Research Applications Laboratory, National Center for Atmospheric Research, Boulder, CO, 80301, USA 4Technical University of Denmark, Copenhagen, 2800, Denmark Correspondence to: Mark W. Seefeldt ([email protected]) Abstract. The Antarctic Precipitation System project deployed and maintained four sites across the northwest Ross Ice Shelf 10 in Antarctica from November 2017 to November 2019. The goals for the project included the collection of in situ observations of precipitation in Antarctica spanning a duration of two years, an improvement in the understanding of precipitation events across the Ross Ice Shelf, and the ability to validate precipitation data from atmospheric numerical models. At each of the four sites the precipitation was measured with an OTT Pluvio2 precipitation gauge. Additionally, snow accumulation at the site was measured with a sonic ranging sensor and using GPS-Interferometry Reflectivity. 15 Supplemental observations of temperature, wind speed, particle count, particle size and speed, and images and video from a camera, were collected to provide context to the precipitation measurements. The collected dataset represents some of the first year-round observations of precipitation in Antarctic at remote locations using an autonomous measurement system. -
RV Investigator Voyage Plan IN2016 V02
RV Investigator Voyage Plan IN2016_V02 SOTS: Southern Ocean Time Series automated moorings for climate and carbon cycle studies southwest of Tasmania CAPRICORN: Clouds, Aerosols, Precipitation, Radiation, and Atmospheric Composition over the Southern Ocean Eddy: Linking eddy physics and biogeochemistry in the Antarctic Circumpolar Current south of Tasmania - 2 - VOYAGE MANAGER Name Tegan Sime Title MNF Operations Officer Email [email protected] PRINCIPAL INVESTIGATOR Name and title Dr Tom Trull, Chief Scientist Affiliation CSIRO Oceans and Atmosphere Contact details [email protected] office: 03 6232 5069 mobile: 04 4779 5735 Name and title Dr. Eric Schulz, Co-Chief Scientist Affiliation Bureau of Meteorology Contact details [email protected] office: 03 9669 4618 ITINERARY Mobilisation: Hobart, Friday-Monday, 11-14 March 2016 Depart: Hobart, ASAP within mobilisation period, targeting Sunday 13 March 10:00, which will allow procedural tests in Storm Bay that day and arrival at SOTS before first light to start mooring deployments. Return: Hobart, Wednesday, 13 April 2015 Targeting 16:00. Demobilisation: Hobart, Thursday, 14 April 2016 IN2015_V01 – Professor Tom Trull - 3 - This voyage combines work from three proposals. The objectives are listed separately for each. Scientific objectives SOTS The Southern Ocean has a predominant role in the movement of heat and carbon dioxide into the ocean interior moderating Earth’s average surface climate. SOTS uses a set of three automated moorings to measure these processes under extreme conditions, where they are most intense and have been least studied. The atmosphere-ocean exchanges occur on many timescales, from daily insolation cycles to ocean basin decadal oscillations and thus high frequency observations sustained over many years are required. -
AWOS) for Initiated By: AJW-144 Change: 1 NON-FEDERAL APPLICATIONS
Advisory Circular U.S. Department of Transportation Federal Aviation Administration Subject: AUTOMATED WEATHER Date: 01/31/2019 AC No: 150/5220-16E OBSERVING SYSTEMS (AWOS) FOR Initiated by: AJW-144 Change: 1 NON-FEDERAL APPLICATIONS 1. PURPOSE. This change contains updates that provide additional clarification and flexibility regarding technician training and responsibilities. These changes expand on the use of on site training or on the job training (OJT), based on statements regarding equivalent performance and maintenance standards in FAA Order 6700.20, Non-Federal Navigational Aids, Air Traffic Control Facilities, and Automated Weather Systems and discussion of on the job training in FAA Order 3000.57, Air Traffic Organization Technical Operations Training and Personnel Certification Programs. 2. PRINCIPAL CHANGES. Changed text is indicated by vertical bars in the margins. The primary revisions are contained in chapter 2, chapter 4 and the addition of appendix 2. Remove Page Date Insert Page Date i 03/10/2017 i 01/31/2019 ii 03/10/2017 ii 01/31/2019 v 03/10/2017 v 01/31/2019 vii 03/10/2017 vii 01/31/2019 8 03/10/2017 8 01/31/2019 12 03/10/2017 12 01/31/2019 18 03/10/2017 18 01/31/2019 64 03/10/2017 64 01/31/2019 A1-1 and A1-2 03/10/2017 A1-1 and A1-2 01/31/2019 A2-1 and A2-2 01/31/2019 David E. Spencer Director Operations Support, AJW-1 01/31/2019 AC 150/5220-16E CHG 1 Advisory Circular U.S.