(ABE) Blended in N-Dodecane for High Pressure-High Temperature Conditions : Application to Compression Ignition Engine Ob Nilaphai
Total Page:16
File Type:pdf, Size:1020Kb
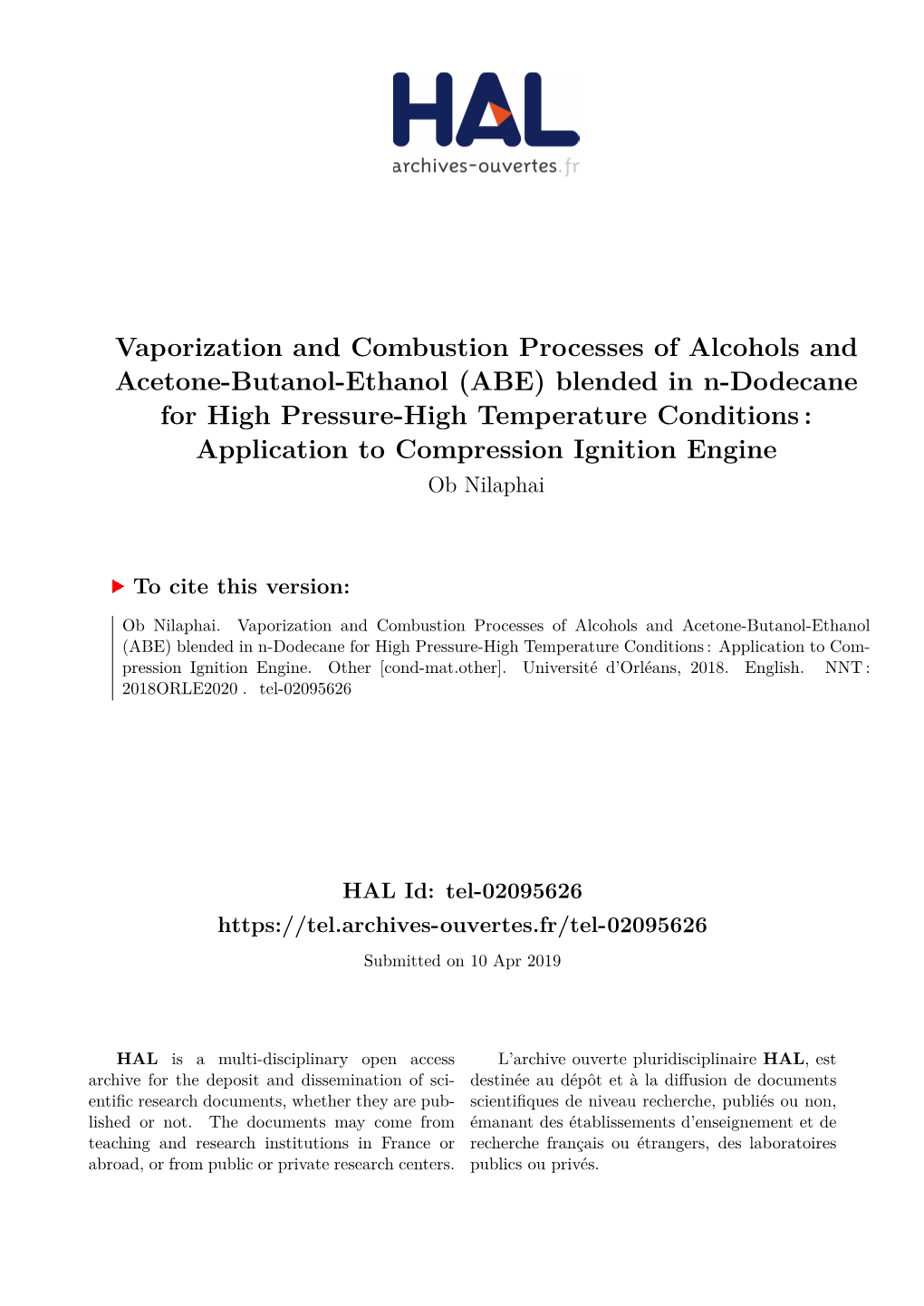
Load more
Recommended publications
-
Surface Tension Study of Concentration Dependent Cluster Breaking in Acetone-Alcohol Systems
www.sciencevision.org Sci Vis 12 (3), 102-105 July-September 2012 Original Research ISSN (print) 0975-6175 ISSN (online) 2229-6026 Surface tension study of concentration dependent cluster breaking in acetone-alcohol systems Jonathan Lalnunsiama* and V. Madhurima Department of Physics, Mizoram University, Aizawl 796 004, India Received 19 April 2012 | Revised 10 May 2012 | Accepted 13 August 2012 ABSTRACT Alcohols are well-known for the formation of clusters due to hydrogen bonds. When a second mo- lecular species such as acetone is added to an alcohol system, the hydrogen bonds are broken lead- ing to a destruction of the molecular clusters. In this paper we report the findings of surface ten- sion study over the entire concentration region of acetone and six alcohol systems over the entire concentration range. The alcohols chosen were methanol, ethanol, isopropanol, butanol, hexanol and octanol. Surface tension was measured using the pendent drop method. Our study showed a specific molecular interaction near the 1:1 concentration of lower alcohols and acetone whereas the higher alcohols did not exhibit the same. Key words: Surface tension; hydrogen bond; alcohols; acetone. INTRODUCTION vent effects4 but such effects are important and hence there are many studies of hydrogen Alcohols are marked by their ability to bonds in solvents.5 Dielectric and calorimetric form hydrogen bonded clusters.1,2 The addi- studies of clusters in alcohols in carbon-tertra- tion of a second molecular species, whether chloride were attributed to homogeneous as- hydrogen-bonded or not, will change the in- sociations of the alcohol.6 For methanol in p- termolecular interactions in the alcohol sys- dioxane the chain-like clusters were seen to tem, especially by breaking of the hydrogen- form the networks and not cyclic structures.7 bonded clusters. -
Toxicological Profile for Acetone Draft for Public Comment
ACETONE 1 Toxicological Profile for Acetone Draft for Public Comment July 2021 ***DRAFT FOR PUBLIC COMMENT*** ACETONE ii DISCLAIMER Use of trade names is for identification only and does not imply endorsement by the Agency for Toxic Substances and Disease Registry, the Public Health Service, or the U.S. Department of Health and Human Services. This information is distributed solely for the purpose of pre dissemination public comment under applicable information quality guidelines. It has not been formally disseminated by the Agency for Toxic Substances and Disease Registry. It does not represent and should not be construed to represent any agency determination or policy. ***DRAFT FOR PUBLIC COMMENT*** ACETONE iii FOREWORD This toxicological profile is prepared in accordance with guidelines developed by the Agency for Toxic Substances and Disease Registry (ATSDR) and the Environmental Protection Agency (EPA). The original guidelines were published in the Federal Register on April 17, 1987. Each profile will be revised and republished as necessary. The ATSDR toxicological profile succinctly characterizes the toxicologic and adverse health effects information for these toxic substances described therein. Each peer-reviewed profile identifies and reviews the key literature that describes a substance's toxicologic properties. Other pertinent literature is also presented, but is described in less detail than the key studies. The profile is not intended to be an exhaustive document; however, more comprehensive sources of specialty information are referenced. The focus of the profiles is on health and toxicologic information; therefore, each toxicological profile begins with a relevance to public health discussion which would allow a public health professional to make a real-time determination of whether the presence of a particular substance in the environment poses a potential threat to human health. -
Dielectric Constant Chart
Dielectric Constants of Common Materials DIELECTRIC MATERIALS DEG. F CONSTANT ABS RESIN, LUMP 2.4-4.1 ABS RESIN, PELLET 1.5-2.5 ACENAPHTHENE 70 3 ACETAL 70 3.6 ACETAL BROMIDE 16.5 ACETAL DOXIME 68 3.4 ACETALDEHYDE 41 21.8 ACETAMIDE 68 4 ACETAMIDE 180 59 ACETAMIDE 41 ACETANILIDE 71 2.9 ACETIC ACID 68 6.2 ACETIC ACID (36 DEGREES F) 36 4.1 ACETIC ANHYDRIDE 66 21 ACETONE 77 20.7 ACETONE 127 17.7 ACETONE 32 1.0159 ACETONITRILE 70 37.5 ACETOPHENONE 75 17.3 ACETOXIME 24 3 ACETYL ACETONE 68 23.1 ACETYL BROMIDE 68 16.5 ACETYL CHLORIDE 68 15.8 ACETYLE ACETONE 68 25 ACETYLENE 32 1.0217 ACETYLMETHYL HEXYL KETONE 66 27.9 ACRYLIC RESIN 2.7 - 4.5 ACTEAL 21 3.6 ACTETAMIDE 4 AIR 1 AIR (DRY) 68 1.000536 ALCOHOL, INDUSTRIAL 16-31 ALKYD RESIN 3.5-5 ALLYL ALCOHOL 58 22 ALLYL BROMIDE 66 7 ALLYL CHLORIDE 68 8.2 ALLYL IODIDE 66 6.1 ALLYL ISOTHIOCYANATE 64 17.2 ALLYL RESIN (CAST) 3.6 - 4.5 ALUMINA 9.3-11.5 ALUMINA 4.5 ALUMINA CHINA 3.1-3.9 ALUMINUM BROMIDE 212 3.4 ALUMINUM FLUORIDE 2.2 ALUMINUM HYDROXIDE 2.2 ALUMINUM OLEATE 68 2.4 1 Dielectric Constants of Common Materials DIELECTRIC MATERIALS DEG. F CONSTANT ALUMINUM PHOSPHATE 6 ALUMINUM POWDER 1.6-1.8 AMBER 2.8-2.9 AMINOALKYD RESIN 3.9-4.2 AMMONIA -74 25 AMMONIA -30 22 AMMONIA 40 18.9 AMMONIA 69 16.5 AMMONIA (GAS?) 32 1.0072 AMMONIUM BROMIDE 7.2 AMMONIUM CHLORIDE 7 AMYL ACETATE 68 5 AMYL ALCOHOL -180 35.5 AMYL ALCOHOL 68 15.8 AMYL ALCOHOL 140 11.2 AMYL BENZOATE 68 5.1 AMYL BROMIDE 50 6.3 AMYL CHLORIDE 52 6.6 AMYL ETHER 60 3.1 AMYL FORMATE 66 5.7 AMYL IODIDE 62 6.9 AMYL NITRATE 62 9.1 AMYL THIOCYANATE 68 17.4 AMYLAMINE 72 4.6 AMYLENE 70 2 AMYLENE BROMIDE 58 5.6 AMYLENETETRARARBOXYLATE 66 4.4 AMYLMERCAPTAN 68 4.7 ANILINE 32 7.8 ANILINE 68 7.3 ANILINE 212 5.5 ANILINE FORMALDEHYDE RESIN 3.5 - 3.6 ANILINE RESIN 3.4-3.8 ANISALDEHYDE 68 15.8 ANISALDOXINE 145 9.2 ANISOLE 68 4.3 ANITMONY TRICHLORIDE 5.3 ANTIMONY PENTACHLORIDE 68 3.2 ANTIMONY TRIBROMIDE 212 20.9 ANTIMONY TRICHLORIDE 166 33 ANTIMONY TRICHLORIDE 5.3 ANTIMONY TRICODIDE 347 13.9 APATITE 7.4 2 Dielectric Constants of Common Materials DIELECTRIC MATERIALS DEG. -
Equilibrium Critical Phenomena in Fluids and Mixtures
: wil Phenomena I Fluids and Mixtures: 'w'^m^ Bibliography \ I i "Word Descriptors National of ac \oo cop 1^ UNITED STATES DEPARTMENT OF COMMERCE • Maurice H. Stans, Secretary NATIONAL BUREAU OF STANDARDS • Lewis M. Branscomb, Director Equilibrium Critical Phenomena In Fluids and Mixtures: A Comprehensive Bibliography With Key-Word Descriptors Stella Michaels, Melville S. Green, and Sigurd Y. Larsen Institute for Basic Standards National Bureau of Standards Washington, D. C. 20234 4. S . National Bureau of Standards, Special Publication 327 Nat. Bur. Stand. (U.S.), Spec. Publ. 327, 235 pages (June 1970) CODEN: XNBSA Issued June 1970 For sale by the Superintendent of Documents, U.S. Government Printing Office, Washington, D.C. 20402 (Order by SD Catalog No. C 13.10:327), Price $4.00. NATtONAL BUREAU OF STAHOAROS AUG 3 1970 1^8106 Contents 1. Introduction i±i^^ ^ 2. Bibliography 1 3. Bibliographic References 182 4. Abbreviations 183 5. Author Index 191 6. Subject Index 207 Library of Congress Catalog Card Number 7O-6O632O ii Equilibrium Critical Phenomena in Fluids and Mixtures: A Comprehensive Bibliography with Key-Word Descriptors Stella Michaels*, Melville S. Green*, and Sigurd Y. Larsen* This bibliography of 1088 citations comprehensively covers relevant research conducted throughout the world between January 1, 1950 through December 31, 1967. Each entry is charac- terized by specific key word descriptors, of which there are approximately 1500, and is indexed both by subject and by author. In the case of foreign language publications, effort was made to find translations which are also cited. Key words: Binary liquid mixtures; critical opalescence; critical phenomena; critical point; critical region; equilibrium critical phenomena; gases; liquid-vapor systems; liquids; phase transitions; ternairy liquid mixtures; thermodynamics 1. -
Acetone-Alcohol, 1:1, Decolorizer Safety Data Sheet According to Federal Register / Vol
Acetone-Alcohol, 1:1, Decolorizer Safety Data Sheet according to Federal Register / Vol. 77, No. 58 / Monday, March 26, 2012 / Rules and Regulations Issue date: 06/16/2014 Revision date: 10/16/2020 Supersedes: 07/30/2020 Version: 2.0 SECTION 1: Identification 1.1. Identification Product form : Mixtures Product name : Acetone-Alcohol, 1:1, Decolorizer Product code : LC10440 1.2. Recommended use and restrictions on use Use of the substance/mixture : For laboratory and manufacturing use only. Recommended use : Laboratory chemicals Restrictions on use : Not for food, drug or household use 1.3. Supplier LabChem, Inc. 1010 Jackson's Pointe Ct. Zelienople, PA 16063 - USA T 412-826-5230 - F 724-473-0647 [email protected] - www.labchem.com 1.4. Emergency telephone number Emergency number : CHEMTREC: 1-800-424-9300 or +1-703-741-5970 SECTION 2: Hazard(s) identification 2.1. Classification of the substance or mixture GHS US classification Flammable liquids Category 2 H225 Highly flammable liquid and vapor Serious eye damage/eye irritation Category 2 H319 Causes serious eye irritation Reproductive toxicity Category 2 H361 Suspected of damaging the unborn child. Specific target organ toxicity (single exposure) Category 1 H370 Causes damage to organs (central nervous system, optic nerve) Specific target organ toxicity (single exposure) Category 3 H336 May cause drowsiness or dizziness Full text of H statements : see section 16 2.2. GHS Label elements, including precautionary statements GHS US labeling Hazard pictograms (GHS US) : Signal word (GHS US) : Danger Hazard statements (GHS US) : H225 - Highly flammable liquid and vapor H319 - Causes serious eye irritation H336 - May cause drowsiness or dizziness H361 - Suspected of damaging the unborn child. -
IR Spectra and Properties of Solid Acetone, an Interstellar and Cometary Molecule
Spectrochimica Acta Part A: Molecular and Biomolecular Spectroscopy 193 (2018) 33–39 Contents lists available at ScienceDirect Spectrochimica Acta Part A: Molecular and Biomolecular Spectroscopy journal homepage: www.elsevier.com/locate/saa IR spectra and properties of solid acetone, an interstellar and cometary molecule Reggie L. Hudson a,⁎, Perry A. Gerakines a, Robert F. Ferrante b a Astrochemistry Laboratory, NASA Goddard Space Flight Center, Greenbelt, MD 20771, USA b Chemistry Department, U.S. Naval Academy, Annapolis, MD 21402, USA article info abstract Article history: Mid-infrared spectra of amorphous and crystalline acetone are presented along with measurements of the refrac- Received 27 September 2017 tive index and density for both forms of the compound. Infrared band strengths are reported for the first time for Received in revised form 21 November 2017 amorphous and crystalline acetone, along with IR optical constants. Vapor pressures and a sublimation enthalpy Accepted 25 November 2017 for crystalline acetone also are reported. Positions of 13C-labeled acetone are measured. Band strengths are com- Available online 29 November 2017 pared to gas-phase values and to the results of a density-functional calculation. A 73% error in previous work is fi Keywords: identi ed and corrected. IR spectroscopy Published by Elsevier B.V. Band strengths Astrochemistry Amorphous solids Ices 1. Introduction cross sections, and an attempt at a mass-balance calculation. Moreover, the phase or state of the authors' acetone ices was not given and there is Among the roughly 200 extraterrestrial molecules identified, there little in the literature with which to compare. are at least two members of nearly every type of common organic com- In the present paper we present new mid-infrared spectra of amor- pound, such as alkanes, alkenes, alkynes, aromatics, alcohols, amines, phous and crystalline acetone along with band strengths and optical nitriles, ethers, epoxides, and mercaptans (thiols). -
List of Surface Tensions and Surface Energies
Surface tension values of some common test liquids for surface energy analysis Name CAS Ref.-No. Surface tension @ 20 °C in mN/m Temperature coefficient in mN/(m K) 1,2-Dichloro ethane 107-06-2 33.30 -0.1428 1,2,3-Tribromo propane 96-11-7 45.40 -0.1267 1,3,5-Trimethylbenzene (Mesitylene) 108-67-8 28.80 -0.0897 1,4-Dioxane 123-91-1 33.00 -0.1391 1,5-Pentanediol 111-29-5 43.30 -0.1161 1-Chlorobutane 109-69-3 23.10 -0.1117 1-Decanol 112-30-1 28.50 -0.0732 1-nitro propane 108-03-2 29.40 -0.1023 1-Octanol 111-87-5 27.60 -0.0795 Acetone (2-Propanone) 67-64-1 25.20 -0.1120 Aniline 22°C (AN) 62-53-3 43.40 -0.1085 2-Aminoethanol 141-43-5 48.89 -0.1115 Anthranilic acid ethylester 22°C 87-25-2 39.30 -0.0935 Anthranilic acid methylester 25 °C 134-20-3 43.71 -0.1152 Benzene 71-43-2 28.88 -0.1291 Benzylalcohol 100-51-6 39.00 -0.0920 Benzylbenzoate (BNBZ) 120-51-4 45.95 -0.1066 Bromobenzene 108-86-1 36.50 -0.1160 Bromoform 75-25-2 41.50 -0.1308 Butyronitrile 109-74-0 28.10 -0.1037 Carbon disulfid 75-15-0 32.30 -0.1484 Quinoline 91-22-5 43.12 -0.1063 Chloro benzene 108-90-7 33.60 -0.1191 Chloroform 67-66-3 27.50 -0.1295 Cyclohexane 110-82-7 24.95 -0.1211 Cyclohexanol 25 °C 108-93-0 34.40 -0.0966 Cyclopentanol 96-41-3 32.70 -0.1011 p-Cymene 99-87-6 28.10 -0.0941 Decalin 493-01-6 31.50 -0.1031 DataPhysics Instruments GmbH • phone + 49 (0)711 77 05 56 0 www.dataphysics-instruments.com • [email protected] Surface tension values of some common test liquids for surface energy analysis Name CAS Ref.-No. -
Acetone-Butanol-Ethanol Fermentation by Engineered Clostridium Beijerinckii and Clostridium Tyrobutyricum
Acetone-Butanol-Ethanol Fermentation by Engineered Clostridium beijerinckii and Clostridium tyrobutyricum DISSERTATION Presented in Partial Fulfillment of the Requirements for the Degree Doctor of Philosophy in the Graduate School of The Ohio State University By Wei-Lun Chang Graduate Program in Food Science and Technology The Ohio State University 2010 Dissertation Committee: Professor Shang-Tian Yang, Advisor Professor Jeffrey Chalmers Professor Ahmed Yousef Copyright by Wei-Lun Chang 2010 Abstract Recently, biofuel production is a popular topic with the rising concerns about the limited supply of fossil fuel and the fluctuating price of crude oil. Biofuels can be categorized into different groups based on their characteristics and applications, including biodiesel, bioalcohols, biogas, and solid biofuels. Bioalcohols, such as methanol, ethanol, and butanol, have been considered to replace transportation fuels. Among the bioalcohols, butanol attracted more and more attentions because of its superiority over other bioalcohol candidates with its similar properties to automobile gasoline, such as octane numbers, energy content, and energy density. Other advantages for using butanol as a biofuel are its low hygroscopicity and low vapor pressure which prevent increase in moisture content in butanol and make butanol a safe and environmentally friendly biofuel. Butanol, a butyl alcohol, can be produced from sugars and starch by solvent- producing clostridia through acetone-butanol-ethanol (ABE) fermentation. ABE fermentation was once a large industrial fermentation for solvent production from starch- based substrates and molasses during the World Wars in the early twentieth century. However, this practice gradually disappeared because its products could not compete with solvents produced through petro-chemical syntheses. -
Estimation of the Refractive Indices of Some Binary Mixtures
Vol. 9(4), pp. 58-64, April, 2015 DOI: 10.5897/AJPAC2015.0613 Article Number: 28B614952528 African Journal of Pure and Applied ISSN 1996 - 0840 Copyright © 2015 Chemistry Author(s) retain the copyright of this article http://www.academicjournals.org/AJPAC Full Length Research Paper Estimation of the refractive indices of some binary mixtures Isehunwa S. O.*, Olanisebe E. B., Ajiboye O. O. and Akintola S. A. Department of Petroleum Engineering, University of Ibadan, Nigeria. Received 4 February, 2015; Accepted 5 March, 2015 Refractive index is a useful fluid characterization parameter with widespread industrial applications. The values for many pure liquids are known or readily available in literature. However, when experimental data are not available, the refractive indices of binary and multi-component liquids are often estimated from the pure components using mixing rules which are sometimes not accurate. This study was designed to measure the refractive indices and evaluate the accuracy of some commonly used mixing rules when applied to benzene-toluene, heptane-hexane, hexane-acetone, heptane-acetic acid and acetic acid-acetone binary mixtures at varying volume fractions and temperatures between 20 and 60°C. A simpler relation based on modified Kay or Arago-Biot mixing rule was demonstrated to have wider range of applicability because of the explicit temperature-dependence term. Key words: Refractive index, mixing rule, binary mixtures, excess volume, refractometer. INTRODUCTION Refractive index is a fundamental physical property which substitutes, additives, and treatment of oils with measures the speed of light in a material and chemicals (Rushton, 1955). Wankhede (2011) used characterizes its optical properties (Singh, 2002). -
Dielectric Constants
Dielectric Constants Common Name Chemical Formula State Degrees F Dielectric Constant (Ethylenedioxy)diethanol-2,2` 68 23.69 ABS Resin 2.4 ABS Resin, lump 2.4-4.1 ABS Resin, pellet Solid 1.5-2.5 Acatic.Acid (36degrees F) Solid 4.1 Acenaphthene C10H6(CH2)2 Solid 70 3 Acetal MeCH(OEt)2 Liquid 70 3.6 Acetal Bromide 16.5 Acetal Doxime 68 3.4 Acetaldehyde C2H4O Liquid 50 21.8 Acetaldehyde C2H4O Liquid 69.8 21.1 Acetaldoxime C2H5ON Liquid 70 3.4 Acetaldoxime C2H5ON Liquid 73.4 3 Acetamide C3H5NO 180 59 Acetamide C3H5NO Liquid 68 41 Acetamide C3H5NO Solid 71.6 4 Acetanilide PhNH-CO-Me Liquid 71 2.9 Acetanilide PhNH-CO-Me Solid 71.6 2.9 Acetic Acid C2H4O2 Liquid 68 6.15 Acetic Acid C2H4O2 Solid 32.5 4.1 Acetic Acid C2H4O2 Liquid 158 6.62 Acetic Acid, Anhydrous 22 Acetic Anhydride H4H6O3 66 21 Acetic Anhydride H4H6O3 Liquid 70 22 Acetoanilide Granules 2.8 Acetone CO-Me2 Liquid 80 20.7 Acetone CO-Me2 Liquid 130 17.7 Acetone CO-Me2 Gas 212 1.015 Acetone CO-Me2 Liquid -112 31 Acetone CO-Me2 32 1.0159 Acetonitrile CH3-CN Liquid 70 37.5 Acetonitrile CH3-CN Liquid 179.6 26.6 Acetophenone C8H8 Liquid 77 17.39 Acetophenone C8H8 Liquid 394 8.64 Acetoxime Me2C:NOH Liquid 75 23.9 Acetoxime Me2C:NOH Liquid 24 3 Acetyl Acetone C5H2O2 Liquid 68 25.7 Acetyl Acetone C5H2O2 68 23.1 Acetyl Bromide Me-COBr Liquid 68 16.5 Acetyl Chloride C2H3ClO Liquid 35.6 16.9 Acetyl Chloride C2H3ClO Liquid 71.6 15.8 Acetyle Acetone 68 25 Acetylene C2H2 Gas 32 1.001 Acetylmethyl Hexyl Ketone Liquid 66 27.9 Acrylic Resin 2.7-6 Acrylonitrile CH2:CH-CN 68 33 Acteal 21.0-3.6 Acteal -
Chemical Engineering Thermodynamics II
Chemical Engineering Thermodynamics II (CHE 303 Course Notes) T.K. Nguyen Chemical and Materials Engineering Cal Poly Pomona (Winter 2009) Contents Chapter 1: Introduction 1.1 Basic Definitions 1-1 1.2 Property 1-2 1.3 Units 1-3 1.4 Pressure 1-4 1.5 Temperature 1-6 1.6 Energy Balance 1-7 Example 1.6-1: Gas in a piston-cylinder system 1-8 Example 1.6-2: Heat Transfer through a tube 1-10 Chapter 2: Thermodynamic Property Relationships 2.1 Type of Thermodynamic Properties 2-1 Example 2.1-1: Electrolysis of water 2-3 Example 2.1-2: Voltage of a hydrogen fuel cell 2-4 2.2 Fundamental Property Relations 2-5 Example 2.2-1: Finding the saturation pressure 2-8 2.3 Equations of State 2-11 2.3-1 The Virial Equation of State 2-11 Example 2.3-1: Estimate the tank pressure 2-12 2.3-2 The Van de Walls Equation of State 2-13 Example 2.3-2: Expansion work with Van de Walls EOS 2-15 2.3-3 Soave-Redlick-Kwong (SRK) Equation 2-17 Example 2.3-3: Gas Pressure with SRK equation 2-17 Example 2.3-4: Volume calculation with SRK equation 2-18 2.4 Properties Evaluations 2-21 Example 2.4-1: Exhaust temperature of a turbine 2-21 Example 2.4-2: Change in temperature with respect to pressure 2-24 Example 2.4-3: Estimation of thermodynamic property 2-26 Example 2.4-4: Heat required to heat a gas 2-27 Chapter 3: Phase Equilibria 3.1 Phase and Pure Substance 3-1 3.2 Phase Behavior 3-4 Example 3.2-1: Specific volume from data 3-7 3.3 Introduction to Phase Equilibrium 3-11 3.4 Pure Species Phase Equilibrium 3-12 3.4-1 Gibbs Free Energy as a Criterion for Chemical Equilibrium -
Combined Theoretical and Experimental Study of Refractive
This is an open access article published under an ACS AuthorChoice License, which permits copying and redistribution of the article or any adaptations for non-commercial purposes. Article pubs.acs.org/JPCB Combined Theoretical and Experimental Study of Refractive Indices of Water−Acetonitrile−Salt Systems † ∥ ‡ ∥ § § ‡ § Ni An, , Bilin Zhuang, , Minglun Li, Yuyuan Lu,*, and Zhen-Gang Wang*, , † College of Chemistry, Jilin University, Changchun 130012, People’s Republic of China ‡ Division of Chemistry and Chemical Engineering, California Institute of Technology, Pasadena, California 91125, United States § State Key Laboratory of Polymer Physics and Chemistry, Changchun Institute of Applied Chemistry, Chinese Academy of Sciences, Changchun 130022, People’s Republic of China *S Supporting Information ABSTRACT: We propose a simple theoretical formula for describing the refractive indices in binary liquid mixtures containing salt ions. Our theory is based on the Clausius−Mossotti equation; it gives the refractive index of the mixture in terms of the refractive indices of the pure liquids and the polarizability of the ionic species, by properly accounting for the volume change upon mixing. The theoretical predictions are tested by extensive experimental measurements of the refractive indices for water−acetonitrile- salt systems for several liquid compositions, different salt species, and a range of salt concentrations. Excellent agreement is obtained in all cases, especially at low salt concentrations, with no fitting parameters. A simplified expression of the refractive index for low salt concentration is also given, which can be the theoretical basis for determination of salt concentration using refractive index measurements. I. INTRODUCTION n = ∑ ϕini The refractive index, n,defined as the ratio of the speed of light i (1) ϕ ∑ in vacuo c0 to the speed of light in the material c, is one of the where the nominal volume fraction i = Vi/ iVi, with Vi most fundamental properties of pure liquids and their solutions denoting the volume of the ith component before mixing.