Transcriptomics Differentiate Two Novel Bioactive Strains of Paenibacillus
Total Page:16
File Type:pdf, Size:1020Kb
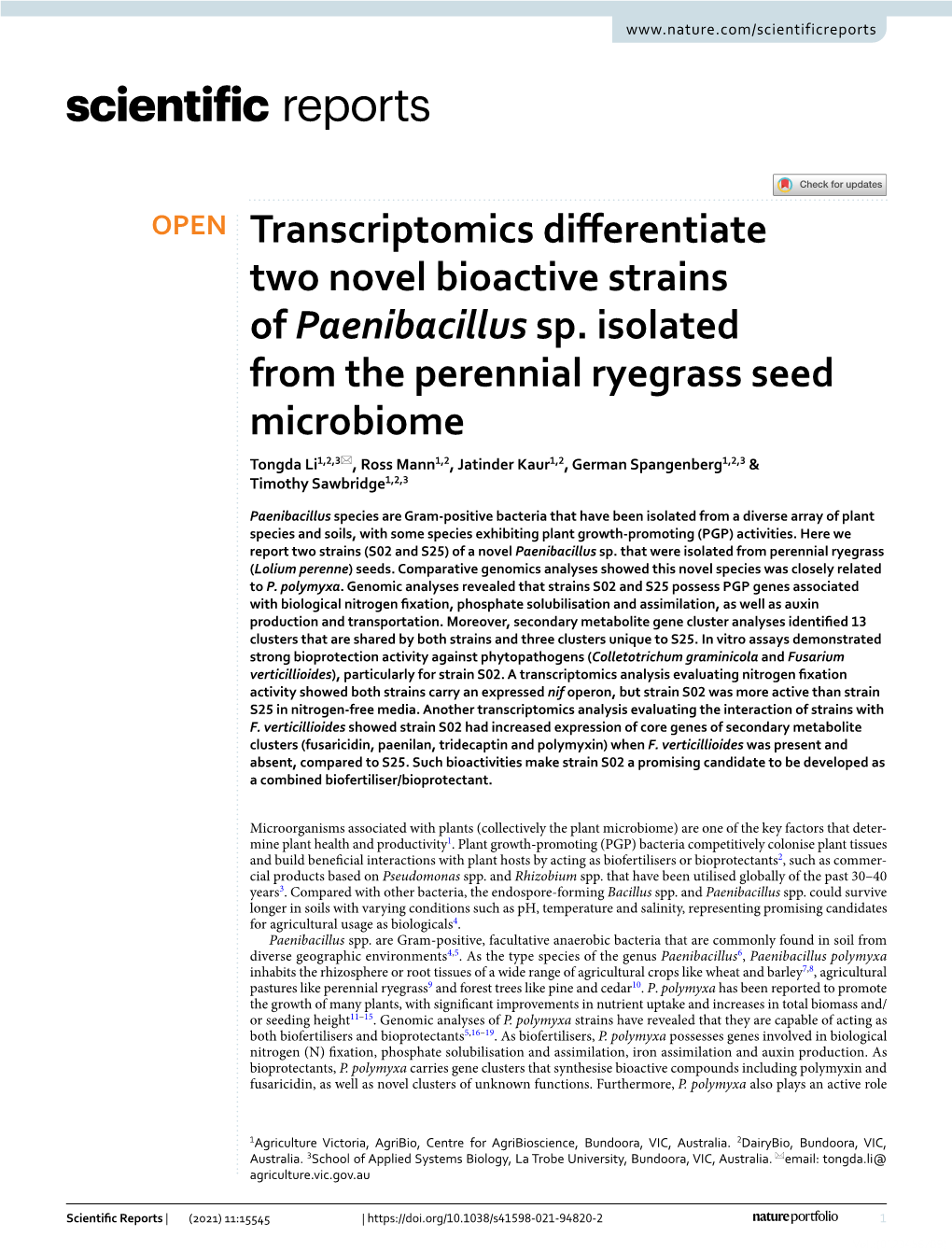
Load more
Recommended publications
-
Relationship Between Sequence Homology, Genome Architecture, and Meiotic Behavior of the Sex Chromosomes in North American Voles
HIGHLIGHTED ARTICLE | INVESTIGATION Relationship Between Sequence Homology, Genome Architecture, and Meiotic Behavior of the Sex Chromosomes in North American Voles Beth L. Dumont,*,1,2 Christina L. Williams,† Bee Ling Ng,‡ Valerie Horncastle,§ Carol L. Chambers,§ Lisa A. McGraw,** David Adams,‡ Trudy F. C. Mackay,*,**,†† and Matthew Breen†,†† *Initiative in Biological Complexity, †Department of Molecular Biomedical Sciences, College of Veterinary Medicine, **Department of Biological Sciences, and ††Comparative Medicine Institute, North Carolina State University, Raleigh, North Carolina 04609, ‡Cytometry Core Facility, Wellcome Sanger Institute, Hinxton, United Kingdom, CB10 1SA and §School of Forestry, Northern Arizona University, Flagstaff, Arizona 86011 ORCID ID: 0000-0003-0918-0389 (B.L.D.) ABSTRACT In most mammals, the X and Y chromosomes synapse and recombine along a conserved region of homology known as the pseudoautosomal region (PAR). These homology-driven interactions are required for meiotic progression and are essential for male fertility. Although the PAR fulfills key meiotic functions in most mammals, several exceptional species lack PAR-mediated sex chromosome associations at meiosis. Here, we leveraged the natural variation in meiotic sex chromosome programs present in North American voles (Microtus) to investigate the relationship between meiotic sex chromosome dynamics and X/Y sequence homology. To this end, we developed a novel, reference-blind computational method to analyze sparse sequencing data from flow- sorted X and Y chromosomes isolated from vole species with sex chromosomes that always (Microtus montanus), never (Microtus mogollonensis), and occasionally synapse (Microtus ochrogaster) at meiosis. Unexpectedly, we find more shared X/Y homology in the two vole species with no and sporadic X/Y synapsis compared to the species with obligate synapsis. -
Molecular Homology and Multiple-Sequence Alignment: an Analysis of Concepts and Practice
CSIRO PUBLISHING Australian Systematic Botany, 2015, 28,46–62 LAS Johnson Review http://dx.doi.org/10.1071/SB15001 Molecular homology and multiple-sequence alignment: an analysis of concepts and practice David A. Morrison A,D, Matthew J. Morgan B and Scot A. Kelchner C ASystematic Biology, Uppsala University, Norbyvägen 18D, Uppsala 75236, Sweden. BCSIRO Ecosystem Sciences, GPO Box 1700, Canberra, ACT 2601, Australia. CDepartment of Biology, Utah State University, 5305 Old Main Hill, Logan, UT 84322-5305, USA. DCorresponding author. Email: [email protected] Abstract. Sequence alignment is just as much a part of phylogenetics as is tree building, although it is often viewed solely as a necessary tool to construct trees. However, alignment for the purpose of phylogenetic inference is primarily about homology, as it is the procedure that expresses homology relationships among the characters, rather than the historical relationships of the taxa. Molecular homology is rather vaguely defined and understood, despite its importance in the molecular age. Indeed, homology has rarely been evaluated with respect to nucleotide sequence alignments, in spite of the fact that nucleotides are the only data that directly represent genotype. All other molecular data represent phenotype, just as do morphology and anatomy. Thus, efforts to improve sequence alignment for phylogenetic purposes should involve a more refined use of the homology concept at a molecular level. To this end, we present examples of molecular-data levels at which homology might be considered, and arrange them in a hierarchy. The concept that we propose has many levels, which link directly to the developmental and morphological components of homology. -
Molecular Homology and Multiple-Sequence Alignment: an Analysis of Concepts and Practice
CSIRO PUBLISHING Australian Systematic Botany, 2015, 28, 46–62 LAS Johnson Review http://dx.doi.org/10.1071/SB15001 Molecular homology and multiple-sequence alignment: an analysis of concepts and practice David A. Morrison A,D, Matthew J. Morgan B and Scot A. Kelchner C ASystematic Biology, Uppsala University, Norbyvägen 18D, Uppsala 75236, Sweden. BCSIRO Ecosystem Sciences, GPO Box 1700, Canberra, ACT 2601, Australia. CDepartment of Biology, Utah State University, 5305 Old Main Hill, Logan, UT 84322-5305, USA. DCorresponding author. Email: [email protected] Abstract. Sequence alignment is just as much a part of phylogenetics as is tree building, although it is often viewed solely as a necessary tool to construct trees. However, alignment for the purpose of phylogenetic inference is primarily about homology, as it is the procedure that expresses homology relationships among the characters, rather than the historical relationships of the taxa. Molecular homology is rather vaguely defined and understood, despite its importance in the molecular age. Indeed, homology has rarely been evaluated with respect to nucleotide sequence alignments, in spite of the fact that nucleotides are the only data that directly represent genotype. All other molecular data represent phenotype, just as do morphology and anatomy. Thus, efforts to improve sequence alignment for phylogenetic purposes should involve a more refined use of the homology concept at a molecular level. To this end, we present examples of molecular-data levels at which homology might be considered, and arrange them in a hierarchy. The concept that we propose has many levels, which link directly to the developmental and morphological components of homology. -
Extensive Intragenic Sequence Homology in Two Distinct Rat Lens Y
Proc. NatL Acad. Sci. USA Vol. 79, pp. 6876-6880, November 1982 Biochemistry Extensive intragenic sequence homology in two distinct rat lens y-crystallin cDNAs suggests duplications of a primordial gene (cDNA cloning/DNA sequence determination/gene generation) ROB J. M. MOORMANN*, JOHAN T. DEN DUNNEN*, HANS BLOEMENDALt, AND JOHN G. G. SCHOENMAKERS* Departments of *Molecular Biology and tBiochemistry, University of Nijmegen, Nijmegen, 6525 ED, The Netherlands Communicated by L. N. M. Duysens, August 17, 1982 ABSTRACT The nucleotide sequences of two different rat formation and intermolecular interactions of the crystallins in lens y-crystallin cDNA clones, pRLy2 and pRLy3, have been the lens fiber cells in situ. However, it has been established that determined. pRLy3 contains the complete coding information for increased light scattering ofsenile nuclear cataract is associated a y-crystallin of 173 amino acids whereas pRLy2 is incomplete in with denaturation, chemical crosslinking including disulfide that it lacks the codons for the first three amino acids ofa separate bridges, and aggregation of crystallins (15). but very homologous y-crystallin of identical length. Both rat y- Recently, the three-dimensional structure of a lens protein, crystallins are homologous to the known amino acid sequence of bovine y-crystallin II, has been determined by Blundell et aL bovine y-crystallin II which is only a single amino acid longer. The (16). It turned out that the sulfhydryl groups in the highly sym- length of the region downstream the coding sequence to the A-A- 3 T-A-A-A polyadenylylation signal sequence is 40 nucleotides in metric two-domain structure of this protein were found in each clone. -
Calculating the Structure-Based Phylogenetic Relationship
CALCULATING THE STRUCTURE-BASED PHYLOGENETIC RELATIONSHIP OF DISTANTLY RELATED HOMOLOGOUS PROTEINS UTILIZING MAXIMUM LIKELIHOOD STRUCTURAL ALIGNMENT COMBINATORICS AND A NOVEL STRUCTURAL MOLECULAR CLOCK HYPOTHESIS A DISSERTATION IN Molecular Biology and Biochemistry and Cell Biology and Biophysics Presented to the Faculty of the University of Missouri-Kansas City in partial fulfillment of the requirements for the degree Doctor of Philosophy by SCOTT GARRETT FOY B.S., Southwest Baptist University, 2005 B.A., Truman State University, 2007 M.S., University of Missouri-Kansas City, 2009 Kansas City, Missouri 2013 © 2013 SCOTT GARRETT FOY ALL RIGHTS RESERVED CALCULATING THE STRUCTURE-BASED PHYLOGENETIC RELATIONSHIP OF DISTANTLY RELATED HOMOLOGOUS PROTEINS UTILIZING MAXIMUM LIKELIHOOD STRUCTURAL ALIGNMENT COMBINATORICS AND A NOVEL STRUCTURAL MOLECULAR CLOCK HYPOTHESIS Scott Garrett Foy, Candidate for the Doctor of Philosophy Degree University of Missouri-Kansas City, 2013 ABSTRACT Dendrograms establish the evolutionary relationships and homology of species, proteins, or genes. Homology modeling, ligand binding, and pharmaceutical testing all depend upon the homology ascertained by dendrograms. Regardless of the specific algorithm, all dendrograms that ascertain protein evolutionary homology are generated utilizing polypeptide sequences. However, because protein structures superiorly conserve homology and contain more biochemical information than their associated protein sequences, I hypothesize that utilizing the structure of a protein instead -
Bioinformatics: a Practical Guide to the Analysis of Genes and Proteins, Second Edition Andreas D
BIOINFORMATICS A Practical Guide to the Analysis of Genes and Proteins SECOND EDITION Andreas D. Baxevanis Genome Technology Branch National Human Genome Research Institute National Institutes of Health Bethesda, Maryland USA B. F. Francis Ouellette Centre for Molecular Medicine and Therapeutics Children’s and Women’s Health Centre of British Columbia University of British Columbia Vancouver, British Columbia Canada A JOHN WILEY & SONS, INC., PUBLICATION New York • Chichester • Weinheim • Brisbane • Singapore • Toronto BIOINFORMATICS SECOND EDITION METHODS OF BIOCHEMICAL ANALYSIS Volume 43 BIOINFORMATICS A Practical Guide to the Analysis of Genes and Proteins SECOND EDITION Andreas D. Baxevanis Genome Technology Branch National Human Genome Research Institute National Institutes of Health Bethesda, Maryland USA B. F. Francis Ouellette Centre for Molecular Medicine and Therapeutics Children’s and Women’s Health Centre of British Columbia University of British Columbia Vancouver, British Columbia Canada A JOHN WILEY & SONS, INC., PUBLICATION New York • Chichester • Weinheim • Brisbane • Singapore • Toronto Designations used by companies to distinguish their products are often claimed as trademarks. In all instances where John Wiley & Sons, Inc., is aware of a claim, the product names appear in initial capital or ALL CAPITAL LETTERS. Readers, however, should contact the appropriate companies for more complete information regarding trademarks and registration. Copyright ᭧ 2001 by John Wiley & Sons, Inc. All rights reserved. No part of this publication may be reproduced, stored in a retrieval system or transmitted in any form or by any means, electronic or mechanical, including uploading, downloading, printing, decompiling, recording or otherwise, except as permitted under Sections 107 or 108 of the 1976 United States Copyright Act, without the prior written permission of the Publisher. -
BLAST Exercise: Detecting and Interpreting Genetic Homology
Last Update: 08/10/2021 BLAST Exercise: Detecting and Interpreting Genetic Homology Adapted by Taylor Cordonnier, Chris Shaffer, Wilson Leung and Sarah C.R. Elgin from Detecting and Interpreting Genetic Homology by Dr. J eremy Buhler Recommended background tutorial An Introduction to NCBI BLAST Resources NCBI BLAST is available at https://blast.ncbi.nlm.nih.gov/Blast.cgi The RepeatMasker web server is available at http://repeatmasker.org/cgi-bin/WEBRepeatMasker The UniProt Protein Knowledgebase is available at https://www.uniprot.org/ Files for this exercise The package containing the files for this exercise is available through the “Detecting and Interpreting Genetic Homology” page on the GEP website. Introduction The Basic Local Alignment Search Tool (BLAST) is a program that reports regions of local similarity (at either the nucleotide or protein level) between a query sequence and sequences within a database. The ability to detect sequence homology allows us to determine if a gene or a protein is related to other known genes or proteins. Detecting sequence homology also facilitates the identification of conserved domains that are shared by multiple genes and the identification of members of a gene family. BLAST is popular because it can efficiently identify regions of local similarity between two sequences. More importantly, BLAST is based on a robust statistical framework. This framework allows BLAST to determine if the alignment between two sequences is statistically significant (i.e. the probability of obtaining an alignment this good by chance is low). Before proceeding with annotation, it is important to understand the inferences that we are making when we use BLAST in our analysis. -
Evolution of the Muscarinic Acetylcholine Receptors in Vertebrates and Dan Larhammar ء,Christina A
New Research Development Evolution of the Muscarinic Acetylcholine Receptors in Vertebrates and Dan Larhammar ء,Christina A. Bergqvist ء,Julia E. Pedersen https://doi.org/10.1523/ENEURO.0340-18.2018 Department of Neuroscience, Unit of Pharmacology, Science for Life Laboratory, Uppsala University, Uppsala SE-751 24, Sweden Abstract The family of muscarinic acetylcholine receptors (mAChRs) consists of five members in mammals, encoded by the CHRM1-5 genes. The mAChRs are G-protein-coupled receptors, which can be divided into the following two subfamilies: M2 and M4 receptors coupling to Gi/o; and M1, M3, and M5 receptors coupling to Gq/11. However, despite the fundamental roles played by these receptors, their evolution in vertebrates has not yet been fully described. We have combined sequence-based phylogenetic analyses with comparisons of exon–intron organi- zation and conserved synteny in order to deduce the evolution of the mAChR receptors. Our analyses verify the existence of two ancestral genes prior to the two vertebrate tetraploidizations (1R and 2R). After these events, one gene had duplicated, resulting in CHRM2 and CHRM4; and the other had triplicated, forming the CHRM1, CHRM3, and CHRM5 subfamily. All five genes are still present in all vertebrate groups investigated except the CHRM1 gene, which has not been identified in some of the teleosts or in chicken or any other birds. Interestingly, the third tetraploidization (3R) that took place in the teleost predecessor resulted in duplicates of all five mAChR genes of which all 10 are present in zebrafish. One of the copies of the CHRM2 and CHRM3 genes and both CHRM4 copies have gained introns in teleosts. -
Nucleic Acids Research
Volufme 9 Number 21 1981 Voum 9 ubr2 91NcecAisRsacNucleic Acids Research Different nuckeotide changes in the hlge rRNA gene of the mitochondrial DNA confer chloramph- enicol resistance on two human cell lnes Hugues Blanc, Camellia W.Adams and Douglas C.Wallace Department of Genetics, Stanford School of Medicine, Stanford, CA 94305, USA Received 3 August 1981 ABSTRACT The nucleotide sequence of the mitochondrial DNA (mtDNA) in the region coding for the 3' end of the large rRNA has been determined for two human cell lines bearing independent cytoplasmic chloramphenicol-resistant (CAP-r) mutations. Comparison of the sequences of these two phenotypically different CAP-r mutants with their CAP-sensitive (CAP-s) parental cell lines has revealed a single base change for each in a region which is highly conserved among species. One CAP-r mutation is associated with an A to G transition on the coding strand while the second contains a G to T transversion 52 nucleotides away. Comparable sequence changes in this region had previously been found for mouse and yeast cell mitochondrial CAP-r mutants. Thus, changes in the large rRNA gene eliminate the inhibition of the ribosome by CAP and different nucleotide changes may result in variations in the drug-r phenotype. INTRODUCTION The human mitochondrial DNA (mtDNA) is 16,569 base pairs (bp) in length and codes for two ribosomal RNAs (rRNAs), 22 transfer RNAs (tRNAs), and 13 polypeptides, 5 of which have been assigned a genetic function (1). Virtually all of the genes including the 12S and 16S rRNA genes are separated by one or more tRNAs. -
Human DNA Sequence Homologous to the Transforming Gene (Mos)
Proc. NatL Acad. Sci. USA Vol. 79, pp. 4078-4082, July 1982 Cell Biology Human DNA sequence homologous to the transforming gene (mos) of Moloney murine sarcoma virus (one genes/mos nucleotide sequence/recombinant DNA/transfection) ROGER WATSON, MARIANNE OSKARSSON, AND GEORGE F. VANDE WOUDE Laboratory of Molecular Oncology, National Cancer Institute, National Institutes of Health, Bethesda, Maryland 20205 Communicated by Paul C. Zamecnik, March 29, 1982 ABSTRACT We describe the molecular cloning of a 9-kilo- (murine)] has revealed only 21-nucleotide and 11-amino-acid base-pairBamHIfragmentfromhumanplacental DNAcontaining differences within 1,111 nucleotides of an open reading frame a sequence homologous to the transforming gene (v-mos) of Mo- codingfor the putative mos-transforminggene product (16). The loney murine sarcoma virus. The DNA sequence of the homolo- transforming potential of the cloned cellular DNA containing gous region of human DNA (termed humos)was resolved andcom- mumos has been tested in DNA transfection assays, and, al- pared to that of the mouse cellular homolog of v-mos (termed though it does not transform fibroblasts by itself, it can be ac- mumos) [Van Beveren, C., van Straaten, F., Galleshaw, J. A. & tivated to transform efficiently by inserting the long terminal Verma, I. M. (1981) Cell 27, 97-108]. The humos gene contained repeat (LTR) ofthe provirus at variable distances 5' to the mu- an open reading frame of 346 codons that was aligned with the equivalent mumos DNA sequence by the introduction oftwo gaps mos gene (2, 3, 6). of 15 and 3bases into the mumos DNA and a single gap of9 bases By molecular hybridization in liquid itwas demonstrated that into the humos DNA. -
Primer on Molecular Genetics
DOE Human Genome Program Primer on Molecular Genetics Date Published: June 1992 U.S. Department of Energy Office of Energy Research Office of Health and Environmental Research Washington, DC 20585 The "Primer on Molecular Genetics" is taken from the June 1992 DOE Human Genome 1991-92 Program Report. The primer is intended to be an introduction to basic principles of molecular genetics pertaining to the genome project. Human Genome Management Information System Oak Ridge National Laboratory 1060 Commerce Park Oak Ridge, TN 37830 Voice: 865/576-6669 Fax: 865/574-9888 E-mail: [email protected] 2 Contents Primer on Molecular Introduction ............................................................................................................. 5 Genetics DNA............................................................................................................................... 6 Genes............................................................................................................................ 7 Revised and expanded Chromosomes ............................................................................................................... 8 by Denise Casey (HGMIS) from the Mapping and Sequencing the Human Genome ...................................... 10 primer contributed by Charles Cantor and Mapping Strategies ..................................................................................................... 11 Sylvia Spengler Genetic Linkage Maps ........................................................................................... -
Nucleotide Sequence of the Cro-Cii-Oop Region of Bacteriophage
Volume 6 Number 3 March 1979 Nucleic Acids Research Nucleotide sequence of the cro - cll - oop region of bacteriophage 434 DNA Rudolf Grosschedl and Elisabeth Schwarz* Institut f'ur Biologie III der Universitat Freiburg, Schlanzlestr. 1, D-7800 Freiburg i. Br., GFR Received 2 January 1979 ABSTRACT The nucleotide sequence of a 869 bp segment of phage 434 DNA in- cluding the regulatory genes cro and cII is presented and compared with the corresponding part of the phage A DNA sequence. The 434 cro protein as deduced from the DNA sequence is a highly basic protein of 71 amino acid residues with a calculated molecular weight of 8089. While the cro gene sequences of phage 434 and X DNA are very different, the nuc- leotide sequences to the right of the XAim434 boundary show differences only at 11 out of 512 positions. Nucleotide substitutions in the cII gene occur with one exception in the third positions of the respective codons and only one out of several DNA regulatory signals located in this region of the phage genomes is affected by these nucleotide sub- stitutions. INTRODUCTION Bacteriophage 434 is genetically very similar to phage X and together with several others belongs to the group of lambdoid coliphages. All of them share as common characteristics comparable DNA lengths and a similar structural and functional organization of their genomes. Genes coding for biologically related functions quite often are clustered into contiguous groups such as the imunity and replication regions. Because of their functional equivalence, these groups are exchangeable among the various lambdoid phages, resulting in the formation of hybrid bacteriophages such as X imm434 (1), i21 (2) and others.