Comparing Source Rock Maturity with Pore Size Distribution and Fluid Saturation in the Bakken-Three Forks Petroleum System, Williston Basin, North Dakota
Total Page:16
File Type:pdf, Size:1020Kb
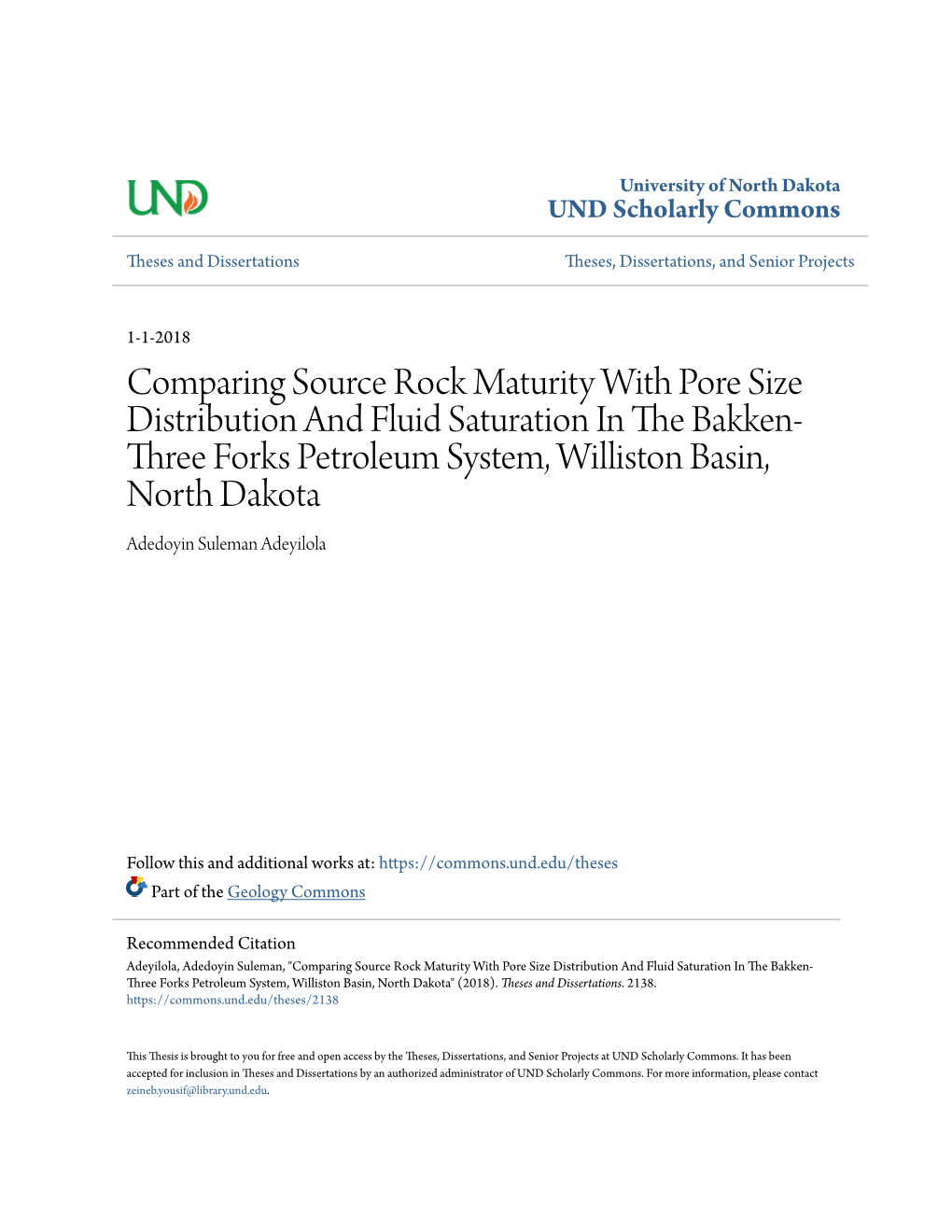
Load more
Recommended publications
-
Case 21-10570-MFW Doc 257 Filed 04/14/21 Page 1 of 127 Case 21-10570-MFW Doc 257 Filed 04/14/21 Page 2 of 127
Case 21-10570-MFW Doc 257 Filed 04/14/21 Page 1 of 127 Case 21-10570-MFW Doc 257 Filed 04/14/21 Page 2 of 127 Exhibit A Case 21-10570-MFW Doc 257 Filed 04/14/21 Page 3 of 127 Exhibit A Served via First-Class Mail Name Attention Address 1 Address 2 Address 3 City State Zip 110 42ND ST WEST LLC 8620 WOLFF CT WESTMINSTER CO 80031 1280 ROYALTIES LLC 5460 S QUEBEC ST STE 335 GREENWOOD VILLAGE CO 80111 13D RESEARCH LLC 6115 ESTATE SMITH BAY BOX 2 SUITE 333 ST THOMAS VI 00802 14 DEZAVALA LLC 14 DEZAVALA PL SAN ANTONIO TX 78231 24 HOUR FLEX & RPS PLAN ADMINISTRATORS P.O. BOX 561552 DENVER CO 80256 26TH STREET LIQUOR INC 105 26TH ST E WILLISTON ND 58801 3 FORKS SERVICES PO BOX 2594 WILLISTON ND 58802 361 SERVICES INC 1401 17TH ST STE 600 DENVER CO 80202 3LAND INC 3101 46TH AVE SE MANDAN ND 58554 42 INTERESTS LLC 2825 BLEDSOE ST FORT WORTH TX 76107 4J MINERAL TRUST DTD 5-25-11 LEONARD W JONES TRUSTEE 6735 59TH ST NW BERTHOLD ND 58718 LOUISE KADANE & MICHAEL L 4K TR PURSUANT DTD 3/1/70 GUSTAFSON TTEE 4809 COLE AVE STE 100 LB 114 DALLAS TX 75205 4TH DIMENSION SURVEYING & CONSULTING INC 14945 COCHISE ST WILLISTON ND 55801 801 LLC PO BOX 900 ARTESIA NM 88211-0900 829 CLINTON INVESTMENTS LLC- CASH SERIES 2 417 LATHROP UNIT 4W RIVER FOREST IL 60305 909 WIRT ROAD PARTNERS 909 WIRT ROAD HOUSTON TX 77024 A & A MARKET INC BOX 1 WILLISTON ND 58802-0001 A C WIENERS A DUANE AHMANN ADDRESS REDACTED A G FERGUSON & ASSOCIATES INC 219 W 11TH HOUSTON TX 77008 A GENE MARTIN FAMILY MINERAL TRUST GENE A AND GENE DAVID MARTIN TT 9021 SUTTERS GOLD DR SACRAMENTO CA 95826 -
Mannville Group of Saskatchewan
Saskatchewan Report 223 Industry and Resources Saskatchewan Geological Survey Jura-Cretaceous Success Formation and Lower Cretaceous Mannville Group of Saskatchewan J.E. Christopher 2003 19 48 Printed under the authority of the Minister of Industry and Resources Although the Department of Industry and Resources has exercised all reasonable care in the compilation, interpretation, and production of this report, it is not possible to ensure total accuracy, and all persons who rely on the information contained herein do so at their own risk. The Department of Industry and Resources and the Government of Saskatchewan do not accept liability for any errors, omissions or inaccuracies that may be included in, or derived from, this report. Cover: Clearwater River Valley at Contact Rapids (1.5 km south of latitude 56º45'; latitude 109º30'), Saskatchewan. View towards the north. Scarp of Middle Devonian Methy dolomite at right. Dolomite underlies the Lower Cretaceous McMurray Formation outcrops recessed in the valley walls. Photo by J.E. Christopher. Additional copies of this digital report may be obtained by contacting: Saskatchewan Industry and Resources Publications 2101 Scarth Street, 3rd floor Regina, SK S4P 3V7 (306) 787-2528 FAX: (306) 787-2527 E-mail: [email protected] Recommended Citation: Christopher, J.E. (2003): Jura-Cretaceous Success Formation and Lower Cretaceous Mannville Group of Saskatchewan; Sask. Industry and Resources, Report 223, CD-ROM. Editors: C.F. Gilboy C.T. Harper D.F. Paterson RnD Technical Production: E.H. Nickel M.E. Opseth Production Editor: C.L. Brown Saskatchewan Industry and Resources ii Report 223 Foreword This report, the first on CD to be released by the Petroleum Geology Branch, describes the geology of the Success Formation and the Mannville Group wherever these units are present in Saskatchewan. -
Nano-Petrophysics Study of the Three Forks Formation in The
NANO-PETROPHYSICS STUDY OF THE THREE FORKS FORMATION IN THE WILLISTON BASIN, NORTH DAKOTA, U.S.A. by DANIEL SAMUEL BAAH Presented to the Faculty of the Graduate School of The University of Texas at Arlington in Partial Fulfillment of the Requirements for the Degree of MASTER OF SCIENCE IN GEOLOGY THE UNIVERSITY OF TEXAS AT ARLINGTON May 2015 Copyright © by Daniel Samuel Baah 2015 All Rights Reserved ii Acknowledgements I would like to begin by thanking God Almighty for His strength, love and grace throughout my research project. Special thanks to my supervisor Dr. Qinhong Hu and my other committee members, Dr. John Wickham and Dr. William Griffith for their support and guidance throughout the project. I would also like to thank the Ms. Julie LeFever and the staff of the Wilson M. Laird Core and Sample Library and the North Dakota Geological Survey for allowing me to use their facilities, photographs, and samples. Lastly, I would like to thank my parents and siblings for their persistent love and support throughout my lifetime of education. Their support in my educational pursuits, their suggestions to improve my work, and also the necessary tools I have needed to succeed in life have ultimately guided me to this point. April 3, 2015 iii Abstract NANO-PETROPHYSICS STUDY OF THE THREE FORKS FORMATION IN THE WILLISTON, NORTH DAKOTA, U.S.A. Daniel Samuel Baah, M.S. The University of Texas at Arlington, 2015 Supervising Professor: Max Qinhong Hu The Late Devonian Three Forks Formation has increasingly become one of the productive plays in the Williston Basin, North Dakota. -
Williston Basin Project (Targeted Geoscience Initiative II): Summary Report on Paleozoic Stratigraphy, Mapping and Hydrocarbon A
Williston Basin Project (Targeted Geoscience Initiative II): Summary report on Paleozoic stratigraphy, mapping and GP2008-2 hydrocarbon assessment, southwestern Manitoba By M.P.B. Nicolas and D. Barchyn GEOSCIENTIFIC PAPER Geoscientific Paper GP2008-2 Williston Basin Project (Targeted Geoscience Initiative II): Summary report on Paleozoic stratigraphy, mapping and hydrocarbon assessment, southwestern Manitoba by M.P.B. Nicolas and D. Barchyn Winnipeg, 2008, reprinted with minor revisions January, 2009 Science, Technology, Energy and Mines Mineral Resources Division Hon. Jim Rondeau John Fox Minister Assistant Deputy Minister John Clarkson Manitoba Geological Survey Deputy Minister E.C. Syme Director ©Queen’s Printer for Manitoba, 2008, reprinted with minor revisions, January 2009 Every possible effort is made to ensure the accuracy of the information contained in this report, but Manitoba Science, Technol- ogy, Energy and Mines does not assume any liability for errors that may occur. Source references are included in the report and users should verify critical information. Any digital data and software accompanying this publication are supplied on the understanding that they are for the sole use of the licensee, and will not be redistributed in any form, in whole or in part, to third parties. Any references to proprietary software in the documentation and/or any use of proprietary data formats in this release do not constitute endorsement by Manitoba Science, Technology, Energy and Mines of any manufacturer’s product. When using information from this publication in other publications or presentations, due acknowledgment should be given to the Manitoba Geological Survey. The following reference format is recommended: Nicolas, M.P.B, and Barchyn, D. -
Conodont Biostratigraphy of the Bakken and Lower Lodgepole Formations (Devonian and Mississippian), Williston Basin, North Dakota Timothy P
University of North Dakota UND Scholarly Commons Theses and Dissertations Theses, Dissertations, and Senior Projects 1986 Conodont biostratigraphy of the Bakken and lower Lodgepole Formations (Devonian and Mississippian), Williston Basin, North Dakota Timothy P. Huber University of North Dakota Follow this and additional works at: https://commons.und.edu/theses Part of the Geology Commons Recommended Citation Huber, Timothy P., "Conodont biostratigraphy of the Bakken and lower Lodgepole Formations (Devonian and Mississippian), Williston Basin, North Dakota" (1986). Theses and Dissertations. 145. https://commons.und.edu/theses/145 This Thesis is brought to you for free and open access by the Theses, Dissertations, and Senior Projects at UND Scholarly Commons. It has been accepted for inclusion in Theses and Dissertations by an authorized administrator of UND Scholarly Commons. For more information, please contact [email protected]. CONODONT BIOSTRATIGRAPHY OF THE BAKKEN AND LOWER LODGEPOLE FORMATIONS (DEVONIAN AND MISSISSIPPIAN), WILLISTON BASIN, NORTH DAKOTA by Timothy P, Huber Bachelor of Arts, University of Minnesota - Morris, 1983 A Thesis Submitted to the Graduate Faculty of the University of North Dakota in partial fulfillment of the requirements for the degree of Master of Science Grand Forks, North Dakota December 1986 This thesis submitted by Timothy P. Huber in partial fulfillment of the requirements for the Degree of Master of Science from the University of North Dakota has been read by the Faculty Advisory Committee under whom the work has been done, and is hereby approved. This thesis meets the standards for appearance and conforms to the style and format requirements of the Graduate School at the University of North Dakota and is hereby approved. -
Preliminary Bulk Density Mapping of the Upper and Lower Bakken Member Shales of Southeastern Saskatchewan: a Potential Indicator for Oil Generation and Expulsion
Preliminary Bulk Density Mapping of the Upper and Lower Bakken Member Shales of Southeastern Saskatchewan: A Potential Indicator for Oil Generation and Expulsion Dan Kohlruss 1 and Kosta Stamatinos 2 Kohlruss, D. and Stamatinos, K. (2014): Preliminary bulk density mapping of the Upper and Lower Bakken Member shales of southeastern Saskatchewan: a potential indicator for oil generation and expulsion; in Summary of Investigations 2014, Volume 1, Saskatchewan Geological Survey, Sask. Ministry of the Economy, Misc. Rep. 2014-4.1, Paper A-2, 7p. Abstract The use of bulk density coupled with formation resistivity of shale source rocks is a possible method of identifying the level of maturity of the rocks, and their potential for oil generation and oil expulsion. The Late Devonian to Early Mississippian Bakken Formation shales of the Williston Basin are extremely rich source rocks and in some areas of the basin have been responsible for generating and expelling vast amounts of oil. Migration of this oil into suitable stratigraphic traps has resulted in unprecedented economic gain in parts of Saskatchewan, Manitoba, North Dakota and Montana. Studies of the Bakken Formation in central Williston Basin in North Dakota have identified areas where its shales are, without question, thermally mature and have generated and expelled oil. However, Bakken Formation shales in northeastern Williston Basin in Saskatchewan have been examined and reported as being thermally immature, with no evidence of oil generation or expulsion. This paper will illustrate how mapping bulk density and formation resistivity has identified anomalous areas in southeast Saskatchewan where Bakken Formation shale source rocks may indeed be thermally mature and may indeed have expelled oil, contrary to previous studies’ findings. -
Jefferson Formation
Nomenclature and Correlation of Lithologic Subdivisions of the Jefferson and Three Forks Formations of Southern Montana and Northern Wyoming By CHARLES A. SANDBERG CONTRIBUTIONS TO STRATIGRAPHY GEOLOGICAL SURVEY BULLETIN 1194-N UNITED STATES GOVERNMENT PRINTING OFFICE, WASHINGTON : 1965 UNITED STATES DEPARTMENT OF THE INTERIOR STEWART L. UDALL, Secretary GEOLOGICAL SURVEY Thomas B. Nolan, Director The U.S. Geological Survey Library catalog card for this publication appears after page N18. For sale by the Superintendent of Documents, U.S. Government Printing Office Washington, D.C., 20402 - Price 15 cents (paper cover) CONTENTS Abstract. ________________ Introduction. _____________ Stratigraphic relations..... Jefferson Formation. ___... Lower member. _______ Birdbear Member. Three Forks Formation. _ _. Logan Gulch Member. Trident Member__.__. Sappington Member. _. References cited.__________ ILLUSTRATIONS Page FIGURE 1. Map showing distribution of members of Jefferson and Three Forks Formations in southern Montana and northern Wyom ing_____________________________________ N2 2. Columnar section of Jefferson Formation at type locality.___. 6 3. Columnar section of Three Forks Formation at type locality.. 8 m CONTRIBUTIONS TO STRATIGRAPHY NOMENCLATURE AND CORRELATION OF LITHOLOGIC SUBDIVISIONS OF THE JEFFERSON AND THREE FORKS FORMATIONS OF SOUTHERN MONTANA AND NORTHERN WYOMING By CHARLES A. SANDBERQ ABSTRACT The Jefferson Formation (Upper Devonian) comprises a thick lower member and the Birdbear Member. The overlying Three Forks Formation (Devonian and Mississippian) comprises the Logan Gulch, Trident, and Sappington Mem bers. These members are described at the classic type section of the Jefferson and Three Forks Formations near Logan, Mont., and their distribution in south ern Montana and northern Wyoming is shown. The members are correlated with stratigraphic units in adjoining parts of Montana, Idaho, Wyoming, North Dakota, and Canada. -
The Letters F and T Refer to Figures Or Tables Respectively
INDEX The letters f and t refer to figures or tables respectively "A" Marker, 312f, 313f Amherstberg Formation, 664f, 728f, 733,736f, Ashville Formation, 368f, 397, 400f, 412, 416, Abitibi River, 680,683, 706 741f, 765, 796 685 Acadian Orogeny, 686, 725, 727, 727f, 728, Amica-Bear Rock Formation, 544 Asiak Thrust Belt, 60, 82f 767, 771, 807 Amisk lowlands, 604 Askin Group, 259f Active Formation, 128f, 132f, 133, 139, 140f, ammolite see aragonite Assiniboia valley system, 393 145 Amsden Group, 244 Assiniboine Member, 412, 418 Adam Creek, Ont., 693,705f Amundsen Basin, 60, 69, 70f Assiniboine River, 44, 609, 637 Adam Till, 690f, 691, 6911,693 Amundsen Gulf, 476, 477, 478 Athabasca, Alta., 17,18,20f, 387,442,551,552 Adanac Mines, 339 ancestral North America miogeocline, 259f Athabasca Basin, 70f, 494 Adel Mountains, 415 Ancient Innuitian Margin, 51 Athabasca mobile zone see Athabasca Adel Mountains Volcanics, 455 Ancient Wall Complex, 184 polymetamorphic terrane Adirondack Dome, 714, 765 Anderdon Formation, 736f Athabasca oil sands see also oil and gas fields, Adirondack Inlier, 711 Anderdon Member, 664f 19, 21, 22, 386, 392, 507, 553, 606, 607 Adirondack Mountains, 719, 729,743 Anderson Basin, 50f, 52f, 359f, 360, 374, 381, Athabasca Plain, 617f Aftonian Interglacial, 773 382, 398, 399, 400, 401, 417, 477f, 478 Athabasca polymetamorphic terrane, 70f, Aguathuna Formation, 735f, 738f, 743 Anderson Member, 765 71-72,73 Aida Formation, 84,104, 614 Anderson Plain, 38, 106, 116, 122, 146, 325, Athabasca River, 15, 20f, 35, 43, 273f, 287f, Aklak -
Devonian Isopach and Structure Maps: Initial Results of the IEA Weyburn CO2 Monitoring and Storage Project Area
Devonian Isopach and Structure Maps: Initial Results of the IEA Weyburn CO2 Monitoring and Storage Project Area L.K. Kreis, P.L. Thomas, R.B. Burke 1, and S.G. Whittaker Kreis, L.K., Thomas, P.L., Burke, R.B., and Whittaker, S.G. (2003): Devonian isopach and structure maps: Initial results of the IEA Weyburn CO2 Monitoring and Storage Project area; in Summary of Investigations 2003, Volume 1, Saskatchewan Geological Survey, Sask. Industry Resources, Misc. Rep. 2003-4.1, CD-ROM, Paper A-5, 6p. Abstract Devonian mapping results from the International Energy Agency’s (IEA) Weyburn CO2 Monitoring and Storage Project have been jointly published by Saskatchewan Industry and Resources and the North Dakota Geological Survey as a test version CD entitled “Devonian Isopach and Structure Maps, IEA Weyburn CO2 Monitoring and Storage Project Area”. This product contains computer-generated regional structural and isopach maps of the Devonian System in Saskatchewan, North Dakota, and Montana. These include the structural and isopach maps of ten formally recognized formations, and isopach maps of six halite (salt) sub-units and four groups. In addition, stratigraphic tops data, a description of the Saskatchewan Land Survey System, and a questionnaire are included. Copies of the CD are available upon request2. Comments and suggestions from this questionnaire will be used to develop future subsurface mapping products from the Petroleum Geology Branch. Keywords: Devonian, subsurface mapping, isopach map, structure map, salt (halite), CO2 storage, Weyburn, southeastern Saskatchewan. 1. Introduction The IEA Weyburn CO2 Monitoring and Storage Project (the Weyburn Project) is a multi-agency investigation into the storage of anthropogenic CO2 in the Mississippian Midale Beds of EnCana Corp.’s Weyburn Pool, southeastern Saskatchewan. -
New Insights Into the Three Forks Group
New insights into the Three Forks Group, southwestern Manitoba: stratigraphic changes and new sedimentological model M.P.B.Nicolas, Manitoba Innovation, Energy and Mines, Manitoba Geological Survey, Winnipeg, MB [email protected] Summary Over the last decade, there has been considerable amount of tight oil exploration and development focus in the Late Devonian-Early Mississippian Three Forks Group of the Williston Basin in southwestern Manitoba, namely the Torquay, Big Valley and Bakken formations. This group has demonstrated remarkable hydrocarbon development capacity, with excellent potential to find new discoveries. Prior to the drilling rush that has ensued, there was little core information to help properly evaluate the Torquay Formation. This formation was previously referred as the Lyleton Formation and the Three Forks Formation of the Qu’Appelle Group in southwestern Manitoba. Detailed evaluation of this formation has resulted in a revision to the stratigraphic nomenclature previously used in Manitoba, by extending eastern Saskatchewan’s current nomenclature into Manitoba. Understanding the stratigraphic framework has also lead to a better understanding of the depositional environment for the Three Forks Group, particularly the Torquay Formation. The depositional environment of the Torquay Formation had received little attention in the past. The first significant attempt was by Christopher (1961) who attributed the oxidation and brecciation of this component units to regional intraformational weathering processes and the development of soil horizons; this model was acceptable for its time, and while still valid today, can be extended into a farther reaching model. The sedimentary model proposed here for the Torquay has no modern analogue, but can best be described as a giant evaporitic platform. -
GAC-MAC Winnipeg 2013 Program with Abstracts
PROGRAM PROGRAMME with abstracts • Pirate Island - Paint Lake by Nancy-lynne Hughes GAC® -MAC volume 36 AGC® -AMC Joint Annual Meeting Congrès annuel conjoint Discover the potential in one of Canada’s most unexplored Archean greenstone gold belts. www.sangold.ca TSX: SGR | OTCQX: SGRCF Located in Eastern Manitoba Welcome! Bienvenue! TABLE OF CONTENTS TABLE DES MATIÈRES ............................................2 Merci aux commanditaires ..............................................2 Welcome to Winnipeg 2013 ...........................................3 Bienvenue à Winnipeg 2013 ...........................................3 Local Organizing Committee .........................................4 Comité organisateur local ...............................................4 Delegate Information .....................................................5 Renseignements généraux ...............................................5 .............................7 Avis aux présentateurs ....................................................7 Convention Centre Floor Plan .......................................8 Plan d’étage du Centre des congrès ................................8 Meeting at a Glance .......................................................9 Aperçu du congrès ..........................................................9 ....................................10 Programme en un coup d’oeil .......................................10 ....................................12 Interventions plénières et particulières .........................12 Special Events -
EMD Shale Gas and Liquids Committee
EMD Shale Gas and Liquids Committee 2016 EMD Shale Gas and Liquids Committee Annual Report Ursula Hammes, Chair Bureau of Economic Geology The University of Texas at Austin May 20, 2016 Vice-Chairs: Vice-Chair: Harris Cander (Industry), BP, Houston, TX Vice-Chair: Sven Egenhoff (University), Colorado State University Vice-Chair: Brian Cardott (Government), Oklahoma Geological Survey, Norman, OK Advisory Group: Kent Bowker, Bowker Petroleum, The Woodlands, TX Brian Cardott, Oklahoma Geological Survey, Norman, OK Peng Li, Arkansas Geological Survey, Little Rock, AK Ken Chew, IHS (retired), Perthsire, Scotland Thomas Chidsey, Utah Geological Survey, Salt Lake City, UT Russell Dubiel, U.S. Geological Survey, Denver, CO Catherine Enomoto, U.S. Geological Survey, Reston, VA William Harrison, Western Michigan University, Kalamazoo, MI Ursula Hammes, Bureau of Economic Geology, Austin, TX Shu Jiang, University of Utah, Salt Lake City, UT Julie LeFever, North Dakota Geological Survey, Grand Forks, ND Jock McCracken, Egret Consulting, Calgary, AB Stephan Nordeng, North Dakota Geological Survey, Grand Forks, ND Rich Nyahay, New York Museum, Albany, NY Stephen Sonnenberg, Colorado School of Mines, Golden, CO Beau Tinnin, Pioneer Natural Resources, TX 1 2016 EMD Shale Gas and Liquids Committee Annual Report EXECUTIVE SUMMARY Shale gas and liquids have been the focus of extensive drilling for the past 10+ years due to improved recovery and high oil and gas prices all over the world. Although there is international interest in exploiting hydrocarbons from these unconventional reservoirs, with active exploration projects on most continents, much of the successful exploitation from shales continues to be in North America, particularly in the United States but increasingly so in Canada.