The Effects of Chronic Alcohol Consumption on the Mouse Endometrium
Total Page:16
File Type:pdf, Size:1020Kb
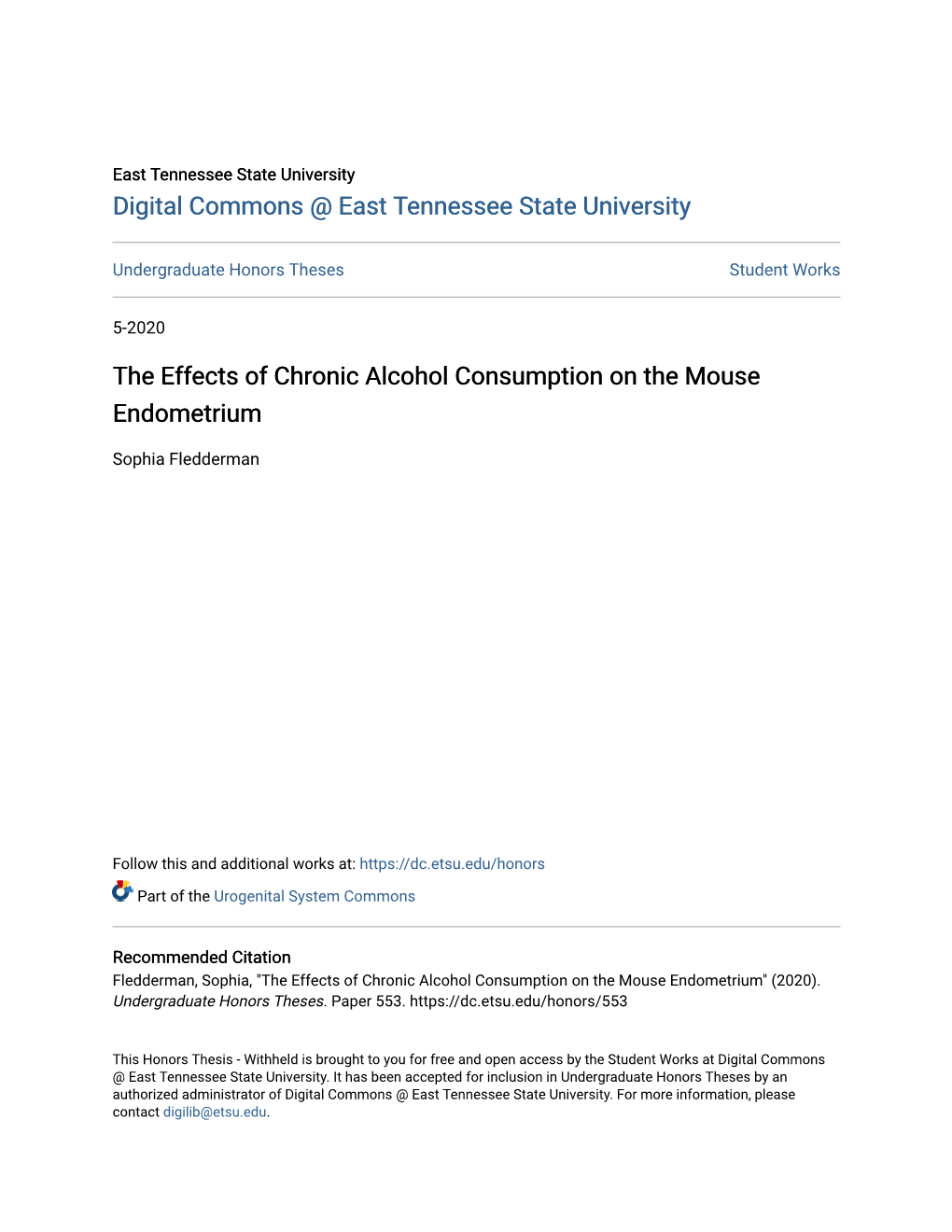
Load more
Recommended publications
-
Human Reproduction and Childbirth
8083DV HUMAN REPRODUCTION AND CHILDBIRTH DVD Version ISBN-13: 978-1-55548-681-5 ISBN: 1-55548-681-9 HUMAN REPRODUCTION AND CHILDBIRTH CREDITS Executive Producer Anson W. Schloat Producer Peter Cochran Script Karin Rhines Teacher’s Resource Book Karin Rhines Former Program Director, Westchester County (NY) Department of Health Copyright 2009 Human Relations Media, Inc. HUMAN RELATIONS MEDIA HUMAN REPRODUCTION AND CHILDBIRTH HUMAN REPRODUCTION AND CHILDBIRTH TABLE OF CONTENTS DVD Menu i Introduction 1 Learning Objectives 2 Program Summary 3 Note to the Teacher 4 Student Activities 1. Pre/Post Test 5 2. Male Anatomy 7 3. Female Anatomy 8 4. Comparative Anatomy 9 5. Matching Quiz 12 6. What Happens When? 14 7. The Fertilization Process 17 8. Care Before Birth 19 9. Research Project 20 10. Being a Parent 22 11. Stem Cells 23 Fact Sheets 1. The Menstrual Cycle 24 2. The Production of Sperm 26 3. Prenatal Care 27 4. Fetal Development 28 5. Screening Newborns for Inherited Diseases 31 6. Prenatal Pictures 32 7. Eating for Two 33 8. Fetal Alcohol Syndrome 35 9. What About Multiples? 36 10. Resources 38 11. Bibliography 39 Other Programs from Human Relations Media 40 HUMAN RELATIONS MEDIA HUMAN REPRODUCTION AND CHILDBIRTH HUMAN REPRODUCTION AND CHILDBIRTH DVD MENU MAIN MENU PLAY CHAPTER SELECTION From here you can access many different paths of the DVD, beginning with the introduction and ending with the credits. 1. Introduction 2. The Male Reproductive System 3. The Female Reproductive System 4. Fertilization and Pregnancy 5. First Trimester 6. Second and Third Trimester 7. -
Chapter V FOLLICULAR DYNAMICS and REPRODUCTIVE
Chapter V FOLLICULAR DYNAMICS AND REPRODUCTIVE TECHNOLOGIES IN BUFFALO Giuseppina Maria Terzano Istituto Sperimentale per la Zootecnia (Animal Production Research Institute) Via Salaria 31, 00016 Monterotondo (Rome), Italy The general characteristics of reproduction like seasonality, cyclicity and ovulation differ widely in mammals for the following reasons: a) reproductive activity may take place during the whole year or at defined seasons, according to the species and their adaptation to environmental conditions; thus, photoperiod plays a determinant role in seasonal breeders such as rodents, carnivores and ruminants (sheep, goats, buffaloes, deer, etc.,). An extreme situation is observed in foxes with only one ovulation per year, occurring in January or February; b) mammals may be distinguished according to the absence or presence of spontaneous ovulations: in the first group of mammals ( rabbits, hares, cats, mink, camels, Llama), the ovulation is induced by mating and cyclicity is not obvious; in the second group, ovulation occurs spontaneously in each cycle, separating the follicular phase from the luteal phase; c) the length of cycles is quite different among species: small rodents have short cycles of four or five days, farm animals and primates have longer cycles (sheep: 17 days; cow, goat, buffalo, horse and pig: 21 days; primates: 28 days), and dogs are characterized by long cycles of six to seven months, including a two month luteal phase (Concannon, 1993); d) ovulation rates differ widely among species and breeds within a given species: in sheep for example, Merinos d'Arles or Ile-de-France breeds have only one ovulation per cycle, whereas average rates of two to four ovulations per cycle are observed in prolific breeds like Romanov or Finn (Land et al., 1973). -
Oogenesis in Mammals
OOGENESIS IN MAMMALS In contrast to most other vertebrates , mammals do not replenish the stores of oocytes present in the ovary at birth. At birth the human ovaries contain about 1 million oocytes ( many of which are already degenerating) that have been arrested in the diplotene stage of the first meiotic division . These oocytes are already surrounded by a layer of follicular cells or granulosa cells , and the complex of ovum and its surrounding cellular investments is known as a follicle . Of all the germ cells present in the ovary ,only about 400 (one per menstrual cycle) will reach maturity and become ovulated. The remainder develop to varying degrees and then undergo atresia (degeneration). Oocytes first become associated with follicular cells in the late fetal period , when they are going through early prophase of the first meiotic division . The primary oocyte (so called because it is undergoing the first meiotic division ) plus its incomplete covering of flattened follicular cells is called a primordial follicle . According to Gougeon (1993) a follicle passes through three major phases on its way to ovulation. The first phase is characterized by a large pool of nongrowing follicles , approximately 500,000 per ovary at birth. In this pool are primordial follicles, which develop into primary follicles by surrounding themselves with a complete single layer of cuboidal follicular cells . By this time , the oocytes have entered the first period of meiotic arrest , the diplotene stage . In human , essentially all oocytes , unless Page 1 of 5 : SEM-2 (GEN ) , Unit#6 : OOGENESIS : Pritha Mondal they degenerate ,remain arrested in the diplotene stage until puberty ; some will not progress past the diplotene stage until the woman’s last reproductive cycle (age 45 to 55 years). -
Knowledge About Human Reproduction and Experience of Puberty 4
KNOWLEDGE ABOUT HUMAN REPRODUCTION AND EXPERIENCE OF PUBERTY 4 4.1 KNOWLEDGE AND EXPERIENCE OF PUBERTY Knowledge of the physiology of human reproduction and the means to protect oneself against sexual or reproductive problems and diseases should be available to adolescents. Better knowledge of these subjects among young adults will lead to correct attitudes and responsible reproductive health behavior. 4.1.1 Knowledge of Physical Changes In the 2002-2003 Indonesia Young Adult Reproductive Health Survey (IYARHS), respondents were asked several questions to measure their knowledge about human reproduction and the experience of puberty. They were asked to name any physical changes that a boy or a girl goes through during the transition from childhood to adolescence. The responses were spontaneous, without any prompting from the interviewer. The findings are presented in Table 4.1. It is interesting to note that while the respondents may have experienced some of the physical changes listed in the questionnaire, some may not have recognized them as part of the process of growing up into adulthood; others may not report them to the interviewer. Table 4.1 Knowledge of physical changes at puberty Percentage of unmarried women and men age 15-24 who know of specific physical changes in a boy and a girl at puberty, by age, IYARHS 2002-2003 Women Men Indicators of physical changes 15-19 20-24 Total 15-19 20-24 Total In a boy Develop muscles 26.3 27.7 26.8 33.1 30.4 32.0 Change in voice 52.2 65.6 56.7 35.5 44.6 39.2 Growth of facial hair, pubic hair, -
The Effectiveness and Safety of the Early Follicular Phase Full-Dose Down
The effectiveness and safety of the early follicular phase full-dose down- regulation protocol for controlled ovarian hyperstimulation: a randomized, paralleled controlled, multicenter trial 2018-12-29 Background Since the first “tube baby”, Louise Brown, was born in the United Kingdom in 1978, many infertile couples have been benefitted from in vitro fertilization and embryo transfer (IVF-ET) and intracytoplasmic sperm injection (ICSI). It is reported that there are over 5 million babies born with the help of assisted reproductive technology (ART). According to the 2015 national data published by Human Fertility and Embryology Authority (HFEA, 48,147 women received 61,726 IVF/ICSI cycles and gave birth to 17,041 newborns [1]. In the United States, 169,602 IVF/ICSI cycles were performed in 2014 and 68,791 tubal babies were born [2]. China has a huge population base, and therefore has a substantial number of infertile couples. Although a late starter, China is developing rapidly in ART and playing a more and more important role in the area of reproductive medicine. In spite of the continuous development in ART, so far, the overall success rate of IVF/ICSI is still hovering around 25-40%. The live birth rate per stimulated cycle is 25.6% in the UK in 2015, fluctuating from 1.9% in women aged 45 and elder to 32.2% in women younger than 35 years old [1]. The IVF/ICSI success rate in 2014 in the US is similar [2]. In China, according to the data submitted by 115 reproductive medicine centers on the ART data reporting system developed by Chinese Society of Reproductive Medicine, the delivery rate is about 40% [3]. -
Luteal Phase Deficiency: What We Now Know
■ OBGMANAGEMENT BY LAWRENCE ENGMAN, MD, and ANTHONY A. LUCIANO, MD Luteal phase deficiency: What we now know Disagreement about the cause, true incidence, and diagnostic criteria of this condition makes evaluation and management difficult. Here, 2 physicians dissect the data and offer an algorithm of assessment and treatment. espite scanty and controversial sup- difficult to definitively diagnose the deficien- porting evidence, evaluation of cy or determine its incidence. Further, while Dpatients with infertility or recurrent reasonable consensus exists that endometrial pregnancy loss for possible luteal phase defi- biopsy is the most reliable diagnostic tool, ciency (LPD) is firmly established in clinical concerns remain about its timing, repetition, practice. In this article, we examine the data and interpretation. and offer our perspective on the role of LPD in assessing and managing couples with A defect of corpus luteum reproductive disorders (FIGURE 1). progesterone output? PD is defined as endometrial histology Many areas of controversy Linconsistent with the chronological date of lthough observational and retrospective the menstrual cycle, based on the woman’s Astudies have reported a higher incidence of LPD in women with infertility and recurrent KEY POINTS 1-4 pregnancy losses than in fertile controls, no ■ Luteal phase deficiency (LPD), defined as prospective study has confirmed these find- endometrial histology inconsistent with the ings. Furthermore, studies have failed to con- chronological date of the menstrual cycle, may be firm the superiority of any particular therapy. caused by deficient progesterone secretion from the corpus luteum or failure of the endometrium Once considered an important cause of to respond appropriately to ovarian steroids. -
Alcohol, Caffeine, and Ivf Success Pesticide Residues
E N V I R O N M E N T A N D R E P R O D U C T I V E H E A L T H ( E A R T H ) S T U D Y N E W S L E T T E R SPRING 2018 | VOL 3 HARVARD T.H. CHAN SCHOOL OF PUBLIC HEALTH, MASSACHUSETTS GENERAL HOSPITAL ALCOHOL, CAFFEINE, AND IVF SUCCESS GREETINGS, Alcohol and caffeine have often been the focus of dietary research We are excited to share our recent findings studies on fertility. Results of these studies have been inconsistent; from the Environment and Reproductive some show benefits while others show no effect or possibly reduced Health (EARTH) Study in our 2018 newsletter! fertility. In the EARTH Study, we found that low to moderate consumption of alcohol and caffeine in the year prior to infertility It has been almost 15 years since the EARTH treatment was not associated with IVF outcomes. Our results suggest Study first began. Thanks to your that women's alcohol intake of less than one alcoholic beverage per participation, we continue to learn more day and caffeine intake below 200mg/day (less than one 12oz cup of about the impact of the environment and coffee per day) in the year prior to IVF did not affect their chances of diet on fertility and pregnancy outcomes successful fertility treatment. We also found that men’s caffeine and among couples recruited from the alcohol consumption did not affect their semen quality (Abadia et al, Massachusetts General Hospital (MGH) Human Reproduction 2017; Karmon et al., Andrology 2017). -
Endocrine Control of Lactational Infertility. I
Maternal Nutrition and Lactational Infertility, edited by I. Dobbing. Nestld Nutrition, Vevey/ Raven Press, New York © 1985. Endocrine Control of Lactational Infertility. I *Alan S. McNeilly, *Anna Glasier, and fPeter W. Howie *MRC Reproductive Biology Unit, Edinburgh EH3 9EW, and 1'Department of Obstetrics and Gynaecology, University of Dundee Medical School, Ninewells Hospital, Dundee DD1 951, Scotland Although there is no doubt that breastfeeding suppresses ovarian activity, the reasons for the immense variability in the duration of this suppression and the mechanisms by which the suckling stimulus causes it remain unclear. The interbirth interval in women who breastfeed can be divided into three main components: (a) the period of lactational amenorrhoea, (b) a period when menstruation returns either during or after lactation, and (c) pregnancy. The length of periods a and b will vary considerably depending on the pattern of breastfeeding, and in a few cases pregnancy will occur during the period of lactational amenorrhoea without an intervening period of menstrual cycles. In an attempt to clarify the mechanisms controlling each of periods a and b above, the changes in endocrine and ovarian activities will be explored. GONADOTROPHIC CONTROL OF THE MENSTRUAL CYCLE Before discussing in detail the influences of suckling on ovarian activity, it is first necessary to outline the basic mechanisms controlling the growth and devel- opment of follicles and subsequent formation of the corpus luteum in the normal menstrual cycle. The basic changes in the four principal hormones involved are shown in Fig. 1. At the time of menses following the demise of the corpus luteum of the previous cycle, follicle development starts, and usually a single follicle begins to grow. -
Variability in the Length of Menstrual Cycles Within and Between Women - a Review of the Evidence Key Points
Variability in the Length of Menstrual Cycles Within and Between Women - A Review of the Evidence Key Points • Mean cycle length ranges from 27.3 to 30.1 days between ages 20 and 40 years, follicular phase length is 13-15 days, and luteal phase length is less variable and averages 13-14 days1-3 • Menstrual cycle lengths vary most widely just after menarche and just before menopause primarily as cycles are anovulatory 1 • Mean length of follicular phase declines with age3,11 while luteal phase remains constant to menopause8 • The variability in menstrual cycle length is attributable to follicular phase length1,11 Introduction Follicular and luteal phase lengths Menstrual cycles are the re-occurring physiological – variability of menstrual cycle changes that happen in women of reproductive age. Menstrual cycles are counted from the first day of attributable to follicular phase menstrual flow and last until the day before the next onset of menses. It is generally assumed that the menstrual cycle lasts for 28 days, and this assumption Key Points is typically applied when dating pregnancy. However, there is variability between and within women with regard to the length of the menstrual cycle throughout • Follicular phase length averages 1,11,12 life. A woman who experiences variations of less than 8 13-15 days days between her longest and shortest cycle is considered normal. Irregular cycles are generally • Luteal phase length averages defined as having 8 to 20 days variation in length of 13-14 days1-3 cycle, whereas over 21 days variation in total cycle length is considered very irregular. -
Infertility Investigations for Women
Infertility investigations for women Brooke Building Gynaecology Department 0161 206 5224 © G21031001W. Design Services, Salford Royal NHS Foundation Trust, All Rights Reserved 2021. Document for issue as handout. Unique Identifier: SURG08(21). Review date: May 2023. This booklet is aimed for women undergoing fertility LH (Luteinising Hormone) Progesterone investigations. Its’ aim is to Oligomenorrhoea - When the provide you with some useful periods are occurring three In women, luteinising hormone Progesterone is a female information regarding your or four times a year (LH) is linked to ovarian hormone produced by the hormone production and egg ovaries after ovulation. It investigations. Irregular cycle - Periods that maturation. LH is used to causes the endometrial lining vary in length We hope you !nd this booklet measure a woman’s ovarian of the uterus to get thicker, helpful. The following blood tests are reserve (egg supply). making it receptive for a used to investigate whether You will be advised to have some It causes the follicles to grow, fertilised egg. ovulation (production of an egg) or all of the following tests: mature and release the eggs Progesterone levels increase is occurring each month and also for fertilisation. It reaches its after ovulation, reaching a to help determine which fertility Hormone blood tests highest level (the LH surge) in maximum level seven days treatments to offer. Follicular bloods tests the middle of the menstrual before the start of the next cycle 48 hours prior to ovulation period. The progesterone test is These routine blood tests are FSH (Follicle Stimulating i.e. days 12-14 of a 28 day cycle. -
Human Reproduction (Chapter- 3)
NOTES HUMAN REPRODUCTION (CHAPTER- 3) Study the following notes along with the N.C.E.R.T Practice all the diagrams in the practice register Refer to the video link at the end of the notes for a better understanding of the chapter Human beings are sexually reproducing, viviparous organisms with internal fertilization and internal development. The reproductive events in human beings include: - Gametogenesis - It is the formation of gametes through the process of meiosis inside the sex organs, that is, testis in males and ovaries in females. Gametes are called as sperms in males and ova or egg in females. - Insemination- It is the transfer of sperms from the male body into the genital tract of female. - Fertilization- It is the fusion of male and female gametes to form a diploid cell called zygote. - Cleavage- The zygote undergoes cleavage that is mitotic divisions to first form a morula and then the blastocyst. - Implantation- The blastocyst gets partially embedded in the walls of the uterus for attachment and nourishment. - Establishment of placenta- A foeto-maternal connective called Placenta develops for attachment, nutrition, respiration and excretion of embryo. - Embryonic development- The implanted embryo undergoes gastrulation (Establishment of the three primary germ layers) and then organogenesis(Development of various tissues, organs and organ systems) - Development and growth of foetus- There is growth of various parts and Systems of the foetus so that they become fully functional. The foetus is then converted into a young one and the period of total stay of embryo in the body of the mother is called gestation period (Pregnancy) - Parturition- The fully formed young one is delivered outside the mother’s body. -
Grade 12 Life Science Human Reproduction Notes
KNOWLEDGE AREA: Life Processes in Plants and Animals TOPIC 2.1: Reproduction in Vertebrates Human Reproduction Introduction Structure of Male Reproductive System Structure of Female Reproductive System Main Changes that occur during Puberty Gametogenesis Menstrual Cycle Fertilization and Embryonic Development Implantation and Development Gestation Role of Placenta There are 2 types of reproduction. These are… 1. Sexual and 2. Asexual reproduction We are studying reproduction in humans. Therefore we need to know what is sexual reproduction. Sexual reproduction is reproduction that occurs with the use of gametes. In humans fertilization occurs during sexual reproduction. This means a haploid sperm fuses with a haploid egg to form a diploid zygote. The zygote has 46 chromosomes or 23 pairs of chromosomes therefore it is called diploid. So how many chromosomes does the egg and sperm have? The sperm has 23 chromosomes The egg has 23 chromosomes The zygote then divides by mitosis to produce a large number of identical cells. All the cells have the same number of chromosomes and identical DNA. Some of these cells become differentiated. This means that the cells undergo physical and chemical changes to perform specialized function. Therefore these cells are adapted for their functions. This is how the body parts are formed. Therefore the zygote eventually develops into a fully formed adult. Sexual maturity occur between 11-15. It is known as puberty. During puberty meiosis occurs in the male and female reproductive organs to produce the gametes. Since the gametes are produced by meiosis, each gamete will have a haploid number of chromosomes and each egg or sperm will be genetically different from the other.