Nonlinear Photoluminescence of Graded-Gap Alxga1 – Xas Solid
Total Page:16
File Type:pdf, Size:1020Kb
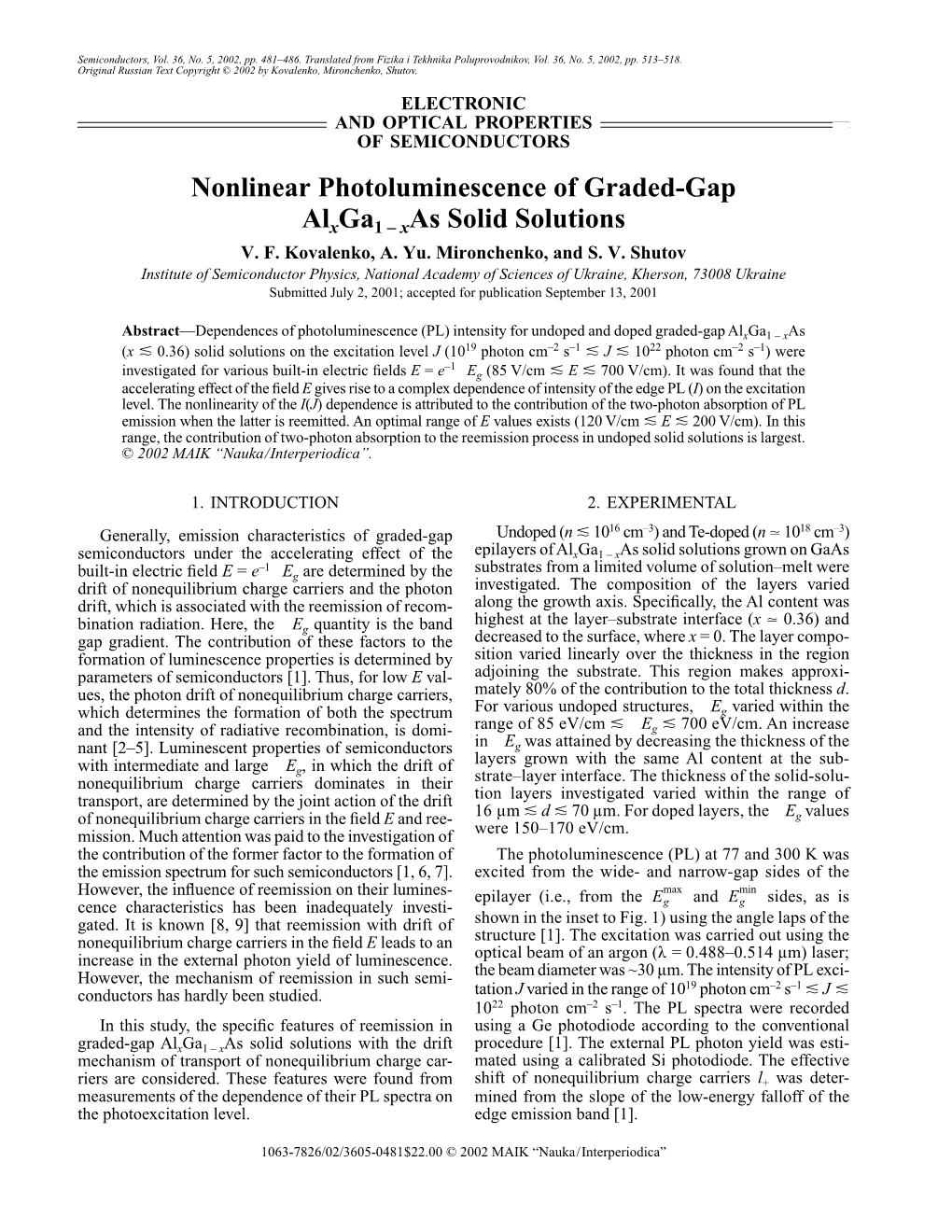
Load more
Recommended publications
-
Gaceta Oficial Del Distrito Federal
GACETA OFICIAL DEL DISTRITO FEDERAL Órgano del Gobierno del Distrito Federal DÉCIMA QUINTA ÉPOCA 21 DE ENERO DE 2005 No. 9 Í N D I C E ADMINISTRACIÓN PÚBLICA DEL DISTRITO FEDERAL SECRETARÍA DE FINANZAS ♦ PARTICIPACIONES ENTREGADAS A LOS ÓRGANOS POLÍTICO-ADMINISTRATIVOS DE LOS FONDOS GENERAL DE PARTICIPACIONES Y DE FOMENTO MUNICIPAL EN EL CUARTO TRIMESTRE DEL AÑO 2004 2 SECRETARÍA DE SEGURIDAD PÚBLICA ♦ RELACIÓN DE PRESTADORES DE SERVICIOS DE SEGURIDAD PRIVADA QUE HAN ACREDITADO DOCUMENTALMENTE LOS REQUISITOS QUE ESTABLECE LA LEY Y REGLAMENTO EN LA MATERIA PARA OBTENER AUTORIZACIÓN Y REGISTRO Y HAN SATISFECHO EL PROCESO DE REVISIÓN 3 DELEGACIÓN COYOACÁN ♦ FE DE ERRATAS 119 CONVOCATORIAS Y LICITACIONES 120 SECCIÓN DE AVISOS ♦ GRUPO COMERCIAL EN COMUNICACIÓN, S.A. DE C.V. 127 ♦ INMOBILIARIA PEDRO DE ALVARADO, S.A. DE C.V. 128 ♦ FIASMEX, S.A. DE C.V. 129 ♦ PIROTE, S.A. 129 ♦ E D I C T O S 130 2 GACETA OFICIAL DE DISTRITO FEDERAL 21 de enero de 2005 ADMINISTRACIÓN PÚBLICA DEL DISTRITO FEDERAL SECRETARÍA DE FINANZAS PARTICIPACIONES ENTREGADAS A LOS ÓRGANOS POLÍTICO-ADMINISTRATIVOS DE LOS FONDOS GENERAL DE PARTICIPACIONES Y DE FOMENTO MUNICIPAL EN EL CUARTO TRIMESTRE DEL AÑO 2004 ARTURO HERRERA GUTIÉRREZ, Secretario de Finanzas del Distrito Federal, con fundamento en los artículos 15, fracción VIII, 16, fracción IV y 30, fracción XIV, de la Ley Orgánica de la Administración Pública del Distrito Federal; 337, 374, 435, 458, 469, fracción I, 478 y 488, del Código Financiero del Distrito Federal; 6°, último párrafo, de la Ley de Coordinación Fiscal; -
Russian Museums Visit More Than 80 Million Visitors, 1/3 of Who Are Visitors Under 18
Moscow 4 There are more than 3000 museums (and about 72 000 museum workers) in Russian Moscow region 92 Federation, not including school and company museums. Every year Russian museums visit more than 80 million visitors, 1/3 of who are visitors under 18 There are about 650 individual and institutional members in ICOM Russia. During two last St. Petersburg 117 years ICOM Russia membership was rapidly increasing more than 20% (or about 100 new members) a year Northwestern region 160 You will find the information aboutICOM Russia members in this book. All members (individual and institutional) are divided in two big groups – Museums which are institutional members of ICOM or are represented by individual members and Organizations. All the museums in this book are distributed by regional principle. Organizations are structured in profile groups Central region 192 Volga river region 224 Many thanks to all the museums who offered their help and assistance in the making of this collection South of Russia 258 Special thanks to Urals 270 Museum creation and consulting Culture heritage security in Russia with 3M(tm)Novec(tm)1230 Siberia and Far East 284 © ICOM Russia, 2012 Organizations 322 © K. Novokhatko, A. Gnedovsky, N. Kazantseva, O. Guzewska – compiling, translation, editing, 2012 [email protected] www.icom.org.ru © Leo Tolstoy museum-estate “Yasnaya Polyana”, design, 2012 Moscow MOSCOW A. N. SCRiAbiN MEMORiAl Capital of Russia. Major political, economic, cultural, scientific, religious, financial, educational, and transportation center of Russia and the continent MUSEUM Highlights: First reference to Moscow dates from 1147 when Moscow was already a pretty big town. -
Ernest Guiraud: a Biography and Catalogue of Works
Louisiana State University LSU Digital Commons LSU Historical Dissertations and Theses Graduate School 1990 Ernest Guiraud: A Biography and Catalogue of Works. Daniel O. Weilbaecher Louisiana State University and Agricultural & Mechanical College Follow this and additional works at: https://digitalcommons.lsu.edu/gradschool_disstheses Recommended Citation Weilbaecher, Daniel O., "Ernest Guiraud: A Biography and Catalogue of Works." (1990). LSU Historical Dissertations and Theses. 4959. https://digitalcommons.lsu.edu/gradschool_disstheses/4959 This Dissertation is brought to you for free and open access by the Graduate School at LSU Digital Commons. It has been accepted for inclusion in LSU Historical Dissertations and Theses by an authorized administrator of LSU Digital Commons. For more information, please contact [email protected]. INFORMATION TO USERS The most advanced technology has been used to photograph and reproduce this manuscript from the microfilm master. UMI films the text directly from the original or copy submitted. Thus, some thesis and dissertation copies are in typewriter face, while others may be from any type of computer printer. The quality of this reproduction is dependent upon the quality of the copy submitted. Broken or indistinct print, colored or poor quality illustrations and photographs, print bleedthrough, substandard margins, and improper alignment can adversely affect reproduction. In the unlikely event that the author did not send UMI a complete manuscript and there are missing pages, these will be noted. Also, if unauthorized copyright material had to be removed, a note will indicate the deletion. Oversize materials (e.g., maps, drawings, charts) are reproduced by sectioning the original, beginning at the upper left-hand corner and continuing from left to right in equal sections with small overlaps. -
Volume 24, Number 04 (April 1906) Winton J
Gardner-Webb University Digital Commons @ Gardner-Webb University The tudeE Magazine: 1883-1957 John R. Dover Memorial Library 4-1-1906 Volume 24, Number 04 (April 1906) Winton J. Baltzell Follow this and additional works at: https://digitalcommons.gardner-webb.edu/etude Part of the Composition Commons, Ethnomusicology Commons, Fine Arts Commons, History Commons, Liturgy and Worship Commons, Music Education Commons, Musicology Commons, Music Pedagogy Commons, Music Performance Commons, Music Practice Commons, and the Music Theory Commons Recommended Citation Baltzell, Winton J.. "Volume 24, Number 04 (April 1906)." , (1906). https://digitalcommons.gardner-webb.edu/etude/513 This Book is brought to you for free and open access by the John R. Dover Memorial Library at Digital Commons @ Gardner-Webb University. It has been accepted for inclusion in The tudeE Magazine: 1883-1957 by an authorized administrator of Digital Commons @ Gardner-Webb University. For more information, please contact [email protected]. APRIL, 1906 ISO PER YEAR ‘TF'TnTT^ PRICE 15 CENTS 180.5 THE ETUDE 209 MODERN SIX-HAND^ LU1T 1 I1 3 Instruction Books PIANO MUSIC “THE ETUDE” - April, 1906 Some Recent Publications Musical Life in New Orleans.. .Alice Graham 217 FOR. THE PIANOFORTE OF «OHE following ensemb Humor in Music. F.S.Law 218 IT styles, and are usi caching purposes t The American Composer. C. von Sternberg 219 CLAYTON F. SUMMY CO. _la- 1 „ net rtf th ’ standard foreign co Experiences of a Music Student in Germany in The following works for beginners at the piano are id some of the lat 1905...... Clarence V. Rawson 220 220 Wabash Avenue, Chicago. -
PIT-FLOOR CRATERS on MERCURY: CHARACTERISTICS and MODES of FORMATION Jeffrey J
40th Lunar and Planetary Science Conference (2009) 2234.pdf PIT-FLOOR CRATERS ON MERCURY: CHARACTERISTICS AND MODES OF FORMATION Jeffrey J. Gillis-Davis1 David T. Blewett2, Brett W. Denevi3, Mark S. Robinson3, Sean C. Solomon4, Robert G. Strom5, and the MESSENGER Team 1Hawaii Institute of Geophysics and Planetology, University of Hawaii, Hono- lulu, HI 96822, [email protected]; 2Johns Hopkins University Applied Physics Laboratory, Laurel, MD 20723, Tempe, AZ 85287; 3School of Earth and Space Exploration, Arizona State University; 4Department of Terrestrial Magnetism, Carnegie Institution of Washington, Washington, DC 20015; 5Lunar and Planetary Laboratory, Univer- sity of Arizona, Tucson, AZ 85721. Introduction: Assessment of volcanic landforms low floors. on Mercury’s surface is important for understanding The juxtaposition of pyroclastic material and pit the planet's thermal history as well as Mercury's place craters suggests that their formation is the result of within the known geologic histories of the other terres- explosive venting of volatiles from degassing magma trial planets [1, 2]. From images obtained by the too deep to propagate to the near surface. A similar MESSENGER spacecraft during its first and second mechanism of formation was suggested for Rima Hy- flyby of Mercury, we have identified numerous pit ginus on the Moon [10]. Calculations of the volatile craters on the floors of impact craters. Pit craters are abundance reveal that magmas on Mercury would re- rimless depressions that are interpreted to have formed quire more than twice the amount of carbon monoxide by endogenic processes. Impact craters hosting pit cra- to emplace pyroclastic deposits a given distance than ters we term pit-floor craters; those identified to date on the Moon [6]. -
Adams Adkinson Aeschlimann Aisslinger Akkermann
BUSCAPRONTA www.buscapronta.com ARQUIVO 27 DE PESQUISAS GENEALÓGICAS 189 PÁGINAS – MÉDIA DE 60.800 SOBRENOMES/OCORRÊNCIA Para pesquisar, utilize a ferramenta EDITAR/LOCALIZAR do WORD. A cada vez que você clicar ENTER e aparecer o sobrenome pesquisado GRIFADO (FUNDO PRETO) corresponderá um endereço Internet correspondente que foi pesquisado por nossa equipe. Ao solicitar seus endereços de acesso Internet, informe o SOBRENOME PESQUISADO, o número do ARQUIVO BUSCAPRONTA DIV ou BUSCAPRONTA GEN correspondente e o número de vezes em que encontrou o SOBRENOME PESQUISADO. Número eventualmente existente à direita do sobrenome (e na mesma linha) indica número de pessoas com aquele sobrenome cujas informações genealógicas são apresentadas. O valor de cada endereço Internet solicitado está em nosso site www.buscapronta.com . Para dados especificamente de registros gerais pesquise nos arquivos BUSCAPRONTA DIV. ATENÇÃO: Quando pesquisar em nossos arquivos, ao digitar o sobrenome procurado, faça- o, sempre que julgar necessário, COM E SEM os acentos agudo, grave, circunflexo, crase, til e trema. Sobrenomes com (ç) cedilha, digite também somente com (c) ou com dois esses (ss). Sobrenomes com dois esses (ss), digite com somente um esse (s) e com (ç). (ZZ) digite, também (Z) e vice-versa. (LL) digite, também (L) e vice-versa. Van Wolfgang – pesquise Wolfgang (faça o mesmo com outros complementos: Van der, De la etc) Sobrenomes compostos ( Mendes Caldeira) pesquise separadamente: MENDES e depois CALDEIRA. Tendo dificuldade com caracter Ø HAMMERSHØY – pesquise HAMMERSH HØJBJERG – pesquise JBJERG BUSCAPRONTA não reproduz dados genealógicos das pessoas, sendo necessário acessar os documentos Internet correspondentes para obter tais dados e informações. DESEJAMOS PLENO SUCESSO EM SUA PESQUISA. -
Finnish Politician. Brought up by an Aunt, He Won An
He wrote two operas, a symphony, two concertos and much piano music, including the notorious Minuet in G (1887). He settled in California in 1913. His international reputation and his efforts for his country P in raising relief funds and in nationalist propaganda during World War I were major factors in influencing Paasikivi, Juho Kusti (originally Johan Gustaf President Woodrow *Wilson to propose the creation Hellsen) (1870–1956). Finnish politician. Brought of an independent Polish state as an Allied war up by an aunt, he won an LLD at Helsinki University, aim. Marshal *Piłsudski appointed Paderewski as becoming an inspector of finances, then a banker. Prime Minister and Foreign Minister (1919) and he Finland declared its independence from Russia represented Poland at the Paris Peace Conference and (1917) and Paasikivi served as Prime Minister 1918, signed the Treaty of Versailles (1919). In December resigning when his proposal for a constitutional he retired and returned to his music but in 1939, monarchy failed. He returned to banking and flirted after Poland had been overrun in World War II, with the semi-Fascist Lapua movement. He was he reappeared briefly in political life as chairman of Ambassador to Sweden 1936–39 and to the USSR the Polish national council in exile. 1939–41. World War II forced him to move from Páez, Juan Antonio (1790–1873). Venezuelan conservatism to realism. *Mannerheim appointed liberator. He fought against the Spanish with varying him Prime Minister 1944–46, and he won two success until he joined (1818) *Bolívar and shared terms as President 1946–56. -
The Life and Death of Professor Alexander P. Borodin: Surgeon, Chemist, and Great Musician
Original communications The life and death of Professor Alexander P. Borodin: Surgeon, chemist, and great musician Igor E. Konstantinov, MD, Charlotte, N.C. From the Department of Thoracic and Cardiovascular Surgery, Carolinas Heart Institute, Charlotte, N.C. Music is one of the most effective and most beautiful means of communication between peoples. However characteristical- ly national the music may be, it will penetrate to the hearts of all receptive listeners regardless of outlook, provided that it has real beauty.1 SEVERAL EMINENT COMPOSERS HAVE flirted with the art of medicine. Hector Berlioz studied medicine in Paris.2,3 Franz von Suppe attended medical school for 1 year before settling down to the more serious work of composing frothy operettas.2 On the other hand, many physicians became more or less proficient in music.4 Perhaps the most promi- nent of them was Theodor Billroth, who became a pianist of high standing.5-7 His lifelong friendship with the famous composer Johannes Brahms, who dedicated his String Quartet, opus 51, to Billroth, is well known.6,7 The two Opus 51 quartets in C minor and A minor have come to be known by musically inclined surgeons as the Billroth I and II.6 However, life is such that very often one thing must be sacrificed in favor of another. For instance, Billroth once invited Brahms to listen to an ama- teur orchestra of physicians. After a few minutes, Brahms stood up and rushed away saying, “No, no, no! I would rather give the Vienna Philharmonic Orchestra to operate on me!” Professor Alexander Borodin combined the roles of medical doctor, chemist, and composer of major rank.8-17 It was my good fortune to study at the Military Fig. -
Classical Music, Propaganda, and the American Cultural Agenda in West Berlin (1945–1949)
Music among the Ruins: Classical Music, Propaganda, and the American Cultural Agenda in West Berlin (1945–1949) by Abby E. Anderton A dissertation submitted in partial fulfillment of the requirements for the degree of Doctor of Philosophy (Music: Musicology) in the University of Michigan 2012 Doctoral Committee: Professor Jane Fair Fulcher, Chair Professor Steven M. Whiting Associate Professor Charles H. Garrett Associate Professor Silke-Maria Weineck To my family ii Acknowledgements While writing this dissertation, I have been so fortunate to have the encouragement of many teachers, friends, and relatives, whose support has been instrumental in this process. My first thanks must go to my wonderful advisor, Dr. Jane Fulcher, and to my committee members, Dr. Charles Garrett, Dean Steven Whiting, and Dr. Silke-Maria Weineck, for their engaging and helpful feedback. Your comments and suggestions were the lifeblood of this dissertation, and I am so grateful for your help. To the life-long friends I made while at Michigan, thank you for making my time in Ann Arbor so enriching, both academically and personally. A thank you to Dennis and to my family, whose constant encouragement has been invaluable. Lastly, I would like to thank my mom and dad, who always encouraged my love of music, even if it meant sitting through eleven community theater productions of The Wizard of Oz. I am more grateful for your help than I could ever express, so I will simply say, “thank you.” iii Table of Contents Dedication ....................................................................................................................... -
CITY MANAGER CITY of CAPE Co~
CITY MANAGER CITY OF CAPE co~. DEPARTMENT OF COMMUNITY D~~~aPMi=tfff 3: ftO MEMORANDUM TO: John Szerlag, City Manager FROM: Vincent A. Cautero, Community Develop~-n~.t Director{!t';)\__, Robert H. Pederson, Planning Manager~ Wyatt Daltry, Planning Team Coordinator vl> DATE: September 6, 2016 SUBJECT: Future Land Use Map Amendment Request-LU16-0012 The City has initiated a large scale future land use map amendment for a large area in Northern Cape Coral; the proposed area is 2,818.49 acres. This request is a follow-up to LU15-0004, which brought over 4,000-acres from the Urban Services Reserve Area into the Urban Services Transition Area. Once the amendment is adopted by Council, property owners could rezone their property for development to permit densities supported by centralized water and sewer utilities. The proposed amendment request includes the following: Current FLU Proposed FLU Acreage Single Family/Multi-Family by PDP (SM) SinQle-Family Residential (SF) 2,686.04 SM Multi-Family Residential (MF) 63.16 SM Parks and Recreation (PK) 10.24 Commercial Activity Center (CAC) SF 29.39 CAC MF 29.66 Thank you for your consideration of this future land use map amendment. Please contact Wyatt Daltry, Planning Team Coordinator, at 573-3160 if you have any questions. VAC/wad(North1 +2FLUMAmemoofintent) Attachment Planning Division Case Report LU 16-0012 Review Date: November 2, 2016 Applicant: City of Cape Coral, Department of Community Development Property Owners: See Attachment A Site Address: See Attachment A Authorized Representative: Wyatt Daltry, AICP Planning Team Coordinator City of Cape Coral Department of Community Development (239) 573-3160 Case Staff: Wyatt Daltry, AICP, Planning Team Coordinator Review Approved By: Robert Pederson, AICP, Planning Manager Purpose: The City has initiated this large-scale future land use map amendment for a large area in Northern Cape Coral. -
National Measurement Laboratory 1979 Technical Highlights
National Measurement laboratory 1 Q7Q Technical Ly,y Highlights U.S. DEPARTMENT OF COMMERCE National Bureau of Standards NBS-SP 572 MATIONAL BU&SAV Or STANDASUS LIBBART JUL 8 1980 National Measurement laboratory 1979 Technical Highlights U.S. DEPARTMENT OF COMMERCE Philip M. Klutznick, Secretary Luther H. Hodges, Jr., Deputy Secretary Jordan J. Baruch, Assistant Secretary for Productivity. Technology and Innovation NATIONAL BUREAU OF STANDARDS Ernest Ambler, Director April 1980 . National Bureau of Standards Special Publication 572 Nat. Bur. Stand. (U.S.), Spec. Publ. 572, 126 pages (April 1980) CODEN: XNBSAV Issued April 1980 U.S. GOVERNMENT PRINTING OFFICE WASHINGTON: 1980 For sale by the Superintendent of Documents, U.S. Government Printing Office, Washington, D.C. 20402 Price $4.25 (Add 25 percent for other than U.S. mailing ) Foreword This report of technical highlights of the National Meas- urement Laboratory is the first in a series of annual re- views. It covers selected scientific accomplishments of the Laboratory for the calendar year 1979. A general Laboratory Overview as well as more specific Center and Program Overviews set the framework for the individual technical reports. These reports represent the wide range of Laboratory activities, the breadth of which should be apparent from the overviews themselves. Future editions of this report will present different, but nevertheless repre- sentative, selections of technical highlights reporting research activities of the preceding year. : , Contents National Measurement Laboratory Overview 1 Center for Thermodynamics and Molecular Science Overview . Center for Absolute Physical Quantities Overview ... 5 39 Bond Energies and Chemical Reactivity Rydberg Values for X- and y-Rays 7 43 Richard D. -
IAU Mercurian Nomenclature
Appendix 1 IAU Mercurian Nomenclature 1. IAU Nomenclature Rules Since its inception in Brussels in 1919 [1], the International Astronomical Union (IAU) has gradually developed a planetary nomenclature system that has evolved from a purely classically based system into a quite so- phisticated attempt to broaden the cultural base of the names approved for planetary bodies and surface features. At present, name selection is guided by 11 rules (quoted verbatim below) in addition to conventions decided upon by nomenclature task groups for individual Solar System bodies. The general rules are as follows1: 1. Nomenclature is a tool and the first consideration should be to make it simple, clear, and unambiguous. 2. In general, official names will not be given to features whose longest di- mensions are less than 100 metres, although exceptions may be made for smaller features having exceptional scientific interest. 3. The number of names chosen for each body should be kept to a minimum. Features should be named only when they have special scientific inter- est, and when the naming of such features is useful to the scientific and cartographic communities at large. 4. Duplication of the same surface feature name on two or more bodies, and of the same name for satellites and minor planets, is discouraged. Duplications may be allowed when names are especially appropriate and the chances for confusion are very small. 5. Individual names chosen for each body should be expressed in the language of origin. Transliteration for various alphabets should be given, but there will be no translation from one language to another.