Comparative Genomics and Genome Evolution in Birds-Of-Paradise
Total Page:16
File Type:pdf, Size:1020Kb
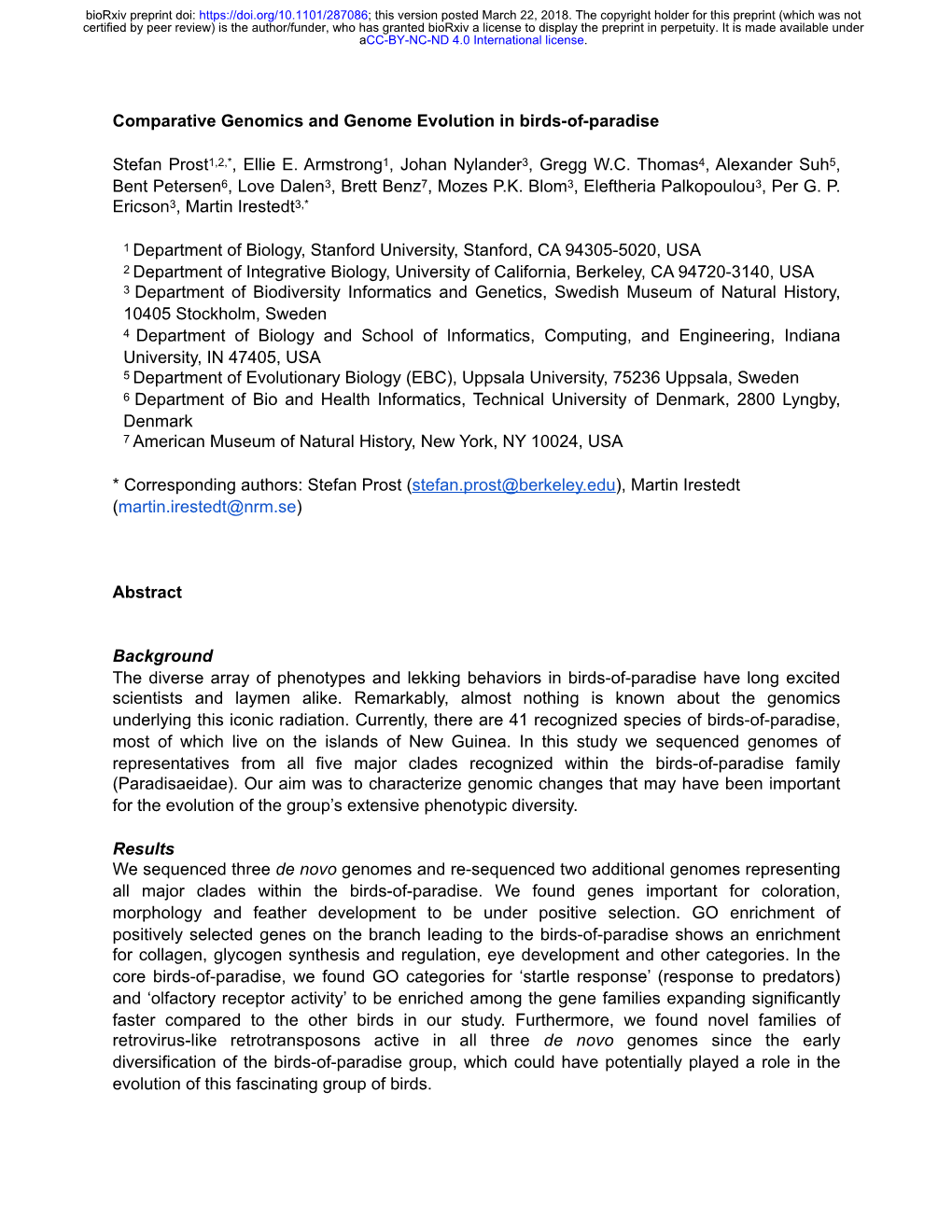
Load more
Recommended publications
-
ORNITHOLOGIST VOLUME 44 - PARTS 1&2 - November - 2019
SOUTH AUSTRALIAN ORNITHOLOGIST VOLUME 44 - PARTS 1&2 - November - 2019 Journal of The South Australian Ornithological Association Inc. In this issue: Variation in songs of the White-eared Honeyeater Phenotypic diversity in the Copperback Quailthrush and a third subspecies Neonicotinoid insecticides Bird Report, 2011-2015: Part 1, Non-passerines President: John Gitsham The South Australian Vice-Presidents: Ornithological John Hatch, Jeff Groves Association Inc. Secretary: Kate Buckley (Birds SA) Treasurer: John Spiers FOUNDED 1899 Journal Editor: Merilyn Browne Birds SA is the trading name of The South Australian Ornithological Association Inc. Editorial Board: Merilyn Browne, Graham Carpenter, John Hatch The principal aims of the Association are to promote the study and conservation of Australian birds, to disseminate the results Manuscripts to: of research into all aspects of bird life, and [email protected] to encourage bird watching as a leisure activity. SAOA subscriptions (e-publications only): Single member $45 The South Australian Ornithologist is supplied to Family $55 all members and subscribers, and is published Student member twice a year. In addition, a quarterly Newsletter (full time Student) $10 reports on the activities of the Association, Add $20 to each subscription for printed announces its programs and includes items of copies of the Journal and The Birder (Birds SA general interest. newsletter) Journal only: Meetings are held at 7.45 pm on the last Australia $35 Friday of each month (except December when Overseas AU$35 there is no meeting) in the Charles Hawker Conference Centre, Waite Road, Urrbrae (near SAOA Memberships: the Hartley Road roundabout). Meetings SAOA c/o South Australian Museum, feature presentations on topics of ornithological North Terrace, Adelaide interest. -
Evolution of Correlated Complexity in the Radically Different Courtship Signals of Birds-Of-Paradise
bioRxiv preprint doi: https://doi.org/10.1101/351437; this version posted June 20, 2018. The copyright holder for this preprint (which was not certified by peer review) is the author/funder, who has granted bioRxiv a license to display the preprint in perpetuity. It is made available under aCC-BY-NC-ND 4.0 International license. Evolution of correlated complexity in the radically different courtship signals of birds-of-paradise 5 Russell A. Ligon1,2*, Christopher D. Diaz1, Janelle L. Morano1, Jolyon Troscianko3, Martin Stevens3, Annalyse Moskeland1†, Timothy G. Laman4, Edwin Scholes III1 1- Cornell Lab of Ornithology, 159 Sapsucker Woods Rd, Ithaca, NY, USA. 10 2- Department of Neurobiology and Behavior, Cornell University, Ithaca, NY 14853, USA. 3- Centre for Ecology and Conservation, College of Life and Environmental Science, University of Exeter, Penryn, Cornwall TR10 9FE, UK 4- Museum of Comparative Zoology, Harvard University, 26 Oxford St., Cambridge, MA 02138, USA 15 † Current address: Department of Zoology, University of Oxford, UK *Author for correspondence: [email protected] ORCID: Russell Ligon https://orcid.org/0000-0002-0195-8275 20 Janelle Morano https://orcid.org/0000-0001-5950-3313 Edwin Scholes https://orcid.org/0000-0001-7724-3201 [email protected] [email protected] 25 [email protected] [email protected] [email protected] [email protected] [email protected] 30 keywords: ornament, complexity, behavioral analyses, sensory ecology, phenotypic radiation 35 1 bioRxiv preprint doi: https://doi.org/10.1101/351437; this version posted June 20, 2018. The copyright holder for this preprint (which was not certified by peer review) is the author/funder, who has granted bioRxiv a license to display the preprint in perpetuity. -
Management and Breeding of Birds of Paradise (Family Paradisaeidae) at the Al Wabra Wildlife Preservation
Management and breeding of Birds of Paradise (family Paradisaeidae) at the Al Wabra Wildlife Preservation. By Richard Switzer Bird Curator, Al Wabra Wildlife Preservation. Presentation for Aviary Congress Singapore, November 2008 Introduction to Birds of Paradise in the Wild Taxonomy The family Paradisaeidae is in the order Passeriformes. In the past decade since the publication of Frith and Beehler (1998), the taxonomy of the family Paradisaeidae has been re-evaluated considerably. Frith and Beehler (1998) listed 42 species in 17 genera. However, the monotypic genus Macgregoria (MacGregor’s Bird of Paradise) has been re-classified in the family Meliphagidae (Honeyeaters). Similarly, 3 species in 2 genera (Cnemophilus and Loboparadisea) – formerly described as the “Wide-gaped Birds of Paradise” – have been re-classified as members of the family Melanocharitidae (Berrypeckers and Longbills) (Cracraft and Feinstein 2000). Additionally the two genera of Sicklebills (Epimachus and Drepanornis) are now considered to be combined as the one genus Epimachus. These changes reduce the total number of genera in the family Paradisaeidae to 13. However, despite the elimination of the 4 species mentioned above, 3 species have been newly described – Berlepsch's Parotia (P. berlepschi), Eastern or Helen’s Parotia (P. helenae) and the Eastern or Growling Riflebird (P. intercedens). The Berlepsch’s Parotia was once considered to be a subspecies of the Carola's Parotia. It was previously known only from four female specimens, discovered in 1985. It was rediscovered during a Conservation International expedition in 2005 and was photographed for the first time. The Eastern Parotia, also known as Helena's Parotia, is sometimes considered to be a subspecies of Lawes's Parotia, but differs in the male’s frontal crest and the female's dorsal plumage colours. -
Nesting Behavior of a Raggiana Bird of Paradise
Wilson Bull., 106(3), 1994, pp. 522-530 NESTING BEHAVIOR OF A RAGGIANA BIRD OF PARADISE WILLIAM E. DAVIS, JR.’ AND BRUCE M. BEEHLER* ABSTRACT..-WC made observations of a nest of a Raggiana Bird of Paradise (Parudisaea raggiana) for 22 days. The single nestling was attended only by the female and was fed only arthropods until day 5, and thereafter a mix of arthropods and fruit. Evidence from regurgitation of seeds at the nest indicates that the parent subsisted largely on fruit. This dietary dichotomy conforms to that of other polygynous birds of paradise and accords with socioecological predictions concerning single-parent nestling care. Received 3 Aug. 1993, accepted 1 Feb. 1994. Many aspects of the life history of birds of paradise (Paradisaeidae) are at least superficially understood (Gilliard 1969, Cooper and Forshaw 1977, Diamond 1981, Beehler 1989). One notable exception is nesting biology which is inadequately documented for many paradisaeid species (Cooper and Forshaw 1977). In spite of recent contributions (Pruett-Jones and Pruett-Jones 1988; Frith and Frith 1990, 1992, 1993a, b; Mack 1992), the nests of 13 species remain undescribed, and 26 species have never been studied at the nest (Cooper and Forshaw 1977; Beehler, unpubl.). Here we provide the first detailed description of nesting behavior of the Raggiana Bird of Paradise (Parudisaea ruggianu) in the wild, one of the best-known members of the family, and Papua New Guinea’s national symbol. The Raggiana Bird of Paradise is a common, vocal, and widespread species of forest and edge that inhabits lowlands and hills of southern, central, and southeastern Papua New Guinea (Cooper and Forshaw 1977). -
(12) United States Patent (10) Patent No.: US 9,605,041 B2 Greengard Et Al
USOO9605041B2 (12) United States Patent (10) Patent No.: US 9,605,041 B2 Greengard et al. (45) Date of Patent: Mar. 28, 2017 (54) REGULATORY PROTEINS AND INHIBITORS 5,385,915 A 1/1995 Buxbaum et al. 5,393,755 A 2f1995 NeuStadt et al. 5,521, 184 A 5/1996 Zimmermann (75) Inventors: Paul Greengard, New York, NY (US); 5,543,520 A 8/1996 Zimmermann Wenjie Luo, New York, NY (US); Gen 5,719,283 A 2f1998 Bell et al. He, New York, NY (US); Peng Li, 5,733,914 A 3/1998 Blankley et al. New York, NY (US); Lawrence 5,744,346 A 4/1998 Chrysler et al. Wennogle, New York, NY (US) 5,777, 195 A 7/1998 Fienberg et al. 5,824,683 A 10, 1998 McKittrick et al. 5,849,770 A 12/1998 Head et al. (73) Assignees: INTRA-CELLULAR THERAPIES, 5,885,834 A 3/1999 Epstein INC., New York, NY (US): THE 5,939,419 A 8, 1999 Tulshian et al. ROCKEFELLER UNIVERSITY, 5,962,492 A 10, 1999 Warrellow New York, NY (US) 6,013,621 A 1/2000 Nishi et al. 6,107.301 A 8, 2000 Aldrich et al. 6,133,273 A 10/2000 Gilbert et al. (*) Notice: Subject to any disclaimer, the term of this 6,147,073. A 11/2000 Battistini et al. patent is extended or adjusted under 35 6,235,742 B1 5, 2001 Bell et al. U.S.C. 154(b) by 974 days. 6,235,746 B1 5, 2001 Davis et al. -
West Papua – Birds-Of-Paradise and Endemics of the Arfaks and Waigeo
INDONESIA: WEST PAPUA – BIRDS-OF-PARADISE AND ENDEMICS OF THE ARFAKS AND WAIGEO 03 – 14 AUGUST 2022 03 – 14 AUGUST 2023 Wilson’s Bird-of-paradise is often considered one of the best-looking birds in the world! www.birdingecotours.com [email protected] 2 | ITINERARY Indonesia: West Papua – Arfak and Waigeo New Guinea is a geographic rather than political term that refers to the main island in the region. The western half of the island of New Guinea comprises the Indonesian provinces of West Papua (Papua Barat) and Papua, collectively once called West Irian or Irian Jaya; the eastern half of the main island of New Guinea comprises the country of Papua New Guinea. We will be based in West Papua for this exhilarating, small-group birding adventure. Aside from the large landmass of New Guinea, the New Guinea region includes numerous small islands (some part of Indonesia and others part of Papua New Guinea), and we will visit one of these areas: Waigeo, part of the Raja Ampat Archipelago in West Papua (also known as the Northwestern Islands). Approximately 680 bird species have been recorded from West Papua, from slightly more than 700 for the whole New Guinea region. Some 550 species are considered breeding residents, with 279 New Guinea endemics (found in Indonesia and/or Papua New Guinea) and at least an additional 42 endemics found only in West Papua. There are also over 115 Palearctic and Australian migrant species and a range of seabirds which spend some of their time in West Papua. This tour will begin in the town of Manokwari, situated on the north-eastern tip of West Papua's Bird's Head (or Vogelkop) Peninsula where we could get our tour started with the gorgeous Lesser Bird-of-paradise, this area is usually great for Blyth’s Hornbill and numerous fruit doves. -
Short Communications Courtship Display and Mating of the Superb
Short Communications Courtship Display and Mating of the Superb Bird of Paradise Lophorina superba D.W. & C.B. FRITH "Prionodura':Paluma via Townsville, Queensland 4816 Emu 88, 183-188 Received 17 May 1987, accepted 9 October 1987 The Superb Bird of Paradise Lophorina superba inhabits December 1977 and November 1985 and we compared rainforest and forest edge between approximately 1000 these with those of the wild birds and descriptions by other and 2250 m above mean sea level (amsl) throughout authors. mainland New Guinea. The adult male is velvet black with metallic-like oil-green iridescent plumage on the crown, an Initial dkplay activily (IDA) erectile iridescent breast shield, and with a very long erectile and spreadable cape of modified velvet black nape This was observed seven times and consists of a sleeked feathers. Peculiar horn-like naral tufts of erectile rather stiff pose, held for about five seconds, before movement of black feathers adorn the base of the upper mandible, above cape, breast and naral tuft plumage. The male slightly the nostrils, as does a similar tuft of feathers beneath the crouches with breast shield sleeked tightly back against lower mandible. The female is cryptically coloured in himself, cape held back and down against the back, wings browns and greys with distinct blackish ventral bamng, and tail held normally, head and bill pointed upwards with like many other female paradisaeids (see Gilliard 1969; eyes fixed on the female, and naral tufts projecting conspic- Cooper & Forshaw 1977). First year male plumage is like uously forward and bifurcate (Fig. 1). This pose is followed that of the female, the black and iridescent plumage of by a repeated, sudden, upward and outward extension of adult males being acquired gradually over several years the breast shield, with head and bill still pointing at the (Gilliard 1969). -
2020 Sample (PDF)
® field guides BIRDING TOURS WORLDWIDE [email protected] • 800•728•4953 ITINERARY NEW GUINEA & AUSTRALIA October 10-28, 2020 One of the most amazing birds in New Guinea, a country full of amazing birds, is the Ribbon-tailed Astrapia. These birds- of-paradise are restricted to a small region in the central highlands. We should see them near Kumul Lodge, although we may not find one with a tail as extravagant as the one pictured here. Photograph by guide Doug Gochfeld. We include here information for those interested in the 2020 Field Guides New Guinea & Australia tour: ¾ a general introduction to the tour ¾ a description of the birding areas to be visited on the tour ¾ an abbreviated daily itinerary with some indication of the nature of each day’s birding outings These additional materials will be made available to those who register for the tour: ¾ an annotated list of the birds recorded on a previous year’s Field Guides trip to the area, with comments by guide(s) on notable species or sightings (may be downloaded from our web site) ¾ a detailed information bulletin with important logistical information and answers to questions regarding accommodations, air arrangements, clothing, currency, customs and immigration, documents, health precautions, and personal items ¾ a reference list ¾ a Field Guides checklist for preparing for and keeping track of the birds we see on the tour ¾ after the conclusion of the tour, a list of birds seen on the tour If you think you may have only one chance to visit Australasia, and you’re looking for a good sampling of the region’s unique wildlife, this tour is designed for you. -
(New Ref)-CG-MS
Send Orders for Reprints to [email protected] 522 Current Genomics, 2018, 19, 522-602 REVIEW ARTICLE Early Life Stress and Epigenetics in Late-onset Alzheimer’s Dementia: A Systematic Review Erwin Lemche* Section of Cognitive Neuropsychiatry, Institute of Psychiatry, Psychology and Neuroscience, King’s College London, London, UK Abstract: Involvement of life stress in Late-Onset Alzheimer’s Disease (LOAD) has been evinced in longitudinal cohort epidemiological studies, and endocrinologic evidence suggests involvements of catecholamine and corticosteroid systems in LOAD. Early Life Stress (ELS) rodent models have suc- cessfully demonstrated sequelae of maternal separation resulting in LOAD-analogous pathology, thereby supporting a role of insulin receptor signalling pertaining to GSK-3beta facilitated tau hyper- phosphorylation and amyloidogenic processing. Discussed are relevant ELS studies, and findings from three mitogen-activated protein kinase pathways (JNK/SAPK pathway, ERK pathway, p38/MAPK pathway) relevant for mediating environmental stresses. Further considered were the roles of auto- phagy impairment, neuroinflammation, and brain insulin resistance. For the meta-analytic evaluation, 224 candidate gene loci were extracted from reviews of animal stud- ies of LOAD pathophysiological mechanisms, of which 60 had no positive results in human LOAD association studies. These loci were combined with 89 gene loci confirmed as LOAD risk genes in A R T I C L E H I S T O R Y previous GWAS and WES. Of the 313 risk gene loci evaluated, there were 35 human reports on epi- Received: July 01, 2017 genomic modifications in terms of methylation or histone acetylation. 64 microRNA gene regulation Revised: July 27, 2017 mechanisms were published for the compiled loci. -
35Th International Society for Animal Genetics Conference 7
35th INTERNATIONAL SOCIETY FOR ANIMAL GENETICS CONFERENCE 7. 23.16 – 7.27. 2016 Salt Lake City, Utah ABSTRACT BOOK https://www.asas.org/meetings/isag2016 INVITED SPEAKERS S0100 – S0124 https://www.asas.org/meetings/isag2016 epigenetic modifications, such as DNA methylation, and measuring different proteins and cellular metab- INVITED SPEAKERS: FUNCTIONAL olites. These advancements provide unprecedented ANNOTATION OF ANIMAL opportunities to uncover the genetic architecture GENOMES (FAANG) ASAS-ISAG underlying phenotypic variation. In this context, the JOINT SYMPOSIUM main challenge is to decipher the flow of biological information that lies between the genotypes and phe- notypes under study. In other words, the new challenge S0100 Important lessons from complex genomes. is to integrate multiple sources of molecular infor- T. R. Gingeras* (Cold Spring Harbor Laboratory, mation (i.e., multiple layers of omics data to reveal Functional Genomics, Cold Spring Harbor, NY) the causal biological networks that underlie complex traits). It is important to note that knowledge regarding The ~3 billion base pairs of the human DNA rep- causal relationships among genes and phenotypes can resent a storage devise encoding information for be used to predict the behavior of complex systems, as hundreds of thousands of processes that can go on well as optimize management practices and selection within and outside a human cell. This information is strategies. Here, we describe a multi-step procedure revealed in the RNAs that are composed of 12 billion for inferring causal gene-phenotype networks underly- nucleotides, considering the strandedness and allelic ing complex phenotypes integrating multi-omics data. content of each of the diploid copies of the genome. -
The Status and Distribution of the Trumpet Manucode Manucodia Keraudrenii (Paradisaeidae) in Australia by CLIFFORD B
AUSTRALIAN 218 BIRD WATCHER AUSTRALIAN BIRD WATCHER 1994, IS, 218-224 The Status and Distribution of the Trumpet Manucode Manucodia keraudrenii (Paradisaeidae) in Australia by CLIFFORD B. FRITH, 'Prionodura', P.O. Box 581, Malanda, Queensland 4885 Summary Early and recent Australian records of the Trumpet Manucode Manucodia keraudrenii south of Coen, on Cape York Peninsula, Queensland, Australia, are reviewed and their validity assessed. No evidence was found that the species is migratory between Papua New Guinea and Australia or that southward vagrant movements occur within Australia, notwithstanding several suggestions to the contrary. The species should be presently treated as a sedentary one, in Australia confined to Cape York Peninsula and several adjacent islands north of Rocky River, Mcllwraith Range, and on Boigu and Saibai Islands adjacent to the Papua New Guinea coast, within Australian territory. Introduction The Trumpet Manucode Manucodia keraudrenii is a large (28 em), crow-like, glossy metallic blue-black bird of paradise which is sexually monomorphic and breeds monogamously. It occurs widely throughout the mountains of mainland New Guinea between 200 and 2000 metres above sea level (Beehler eta!. 1986) , in some lowland areas, on some northernmost islands of the Torres Strait adjacent to Papua New Guinea and on the Aru Islands (Coates 1990). In Australia the species is considered by most authorities to be confined to northern Cape York Peninsula, north of Coen, including adjacent Albany and Mai Islands, and on Boigu and Saibai Islands adjacent to the Papua New Guinea coast (Gilliard 1969, Cooper & Forshaw 1fJ77, Pizzey 1980, Storr 1984, Blakers et a!. 1984, Schodde & Tidemann 1988, Wieneke 1992). -
An Unexpectedly Long History of Sexual Selection in Birds-Of-Paradise
An unexpectedly long history of sexual selection in birds-of-paradise Irestedt, M.; Jønsson, Knud Andreas; Fjeldså, Jon; Christidis, L.; Ericson, P.G.P. Published in: BMC Evolutionary Biology DOI: 10.1186/1471-2148-9-235 Publication date: 2009 Document version Publisher's PDF, also known as Version of record Citation for published version (APA): Irestedt, M., Jønsson, K. A., Fjeldså, J., Christidis, L., & Ericson, P. G. P. (2009). An unexpectedly long history of sexual selection in birds-of-paradise. BMC Evolutionary Biology, 9(235). https://doi.org/10.1186/1471-2148-9- 235 Download date: 28. Sep. 2021 BMC Evolutionary Biology BioMed Central Research article Open Access An unexpectedly long history of sexual selection in birds-of-paradise Martin Irestedt*1, Knud A Jønsson2, Jon Fjeldså2, Les Christidis3,4 and Per GP Ericson1 Address: 1Molecular Systematics Laboratory, Swedish Museum of Natural History, P.O. Box 50007, SE-104 05 Stockholm, Sweden, 2Vertebrate Department, Zoological Museum, University of Copenhagen, Universitetsparken 15, DK-2100 Copenhagen Ø, Denmark, 3Division of Research and Collections, Australian Museum, 6 College St, Sydney, New South Wales 2010, Australia and 4Department of Genetics, University of Melbourne, Parkville, Victoria 3052, Australia Email: Martin Irestedt* - [email protected]; Knud A Jønsson - [email protected]; Jon Fjeldså - [email protected]; Les Christidis - [email protected]; Per GP Ericson - [email protected] * Corresponding author Published: 16 September 2009 Received: 15 May 2009 Accepted: 16 September 2009 BMC Evolutionary Biology 2009, 9:235 doi:10.1186/1471-2148-9-235 This article is available from: http://www.biomedcentral.com/1471-2148/9/235 © 2009 Irestedt et al; licensee BioMed Central Ltd.