Mini and Micro Propulsion for Medical Swimmers
Total Page:16
File Type:pdf, Size:1020Kb
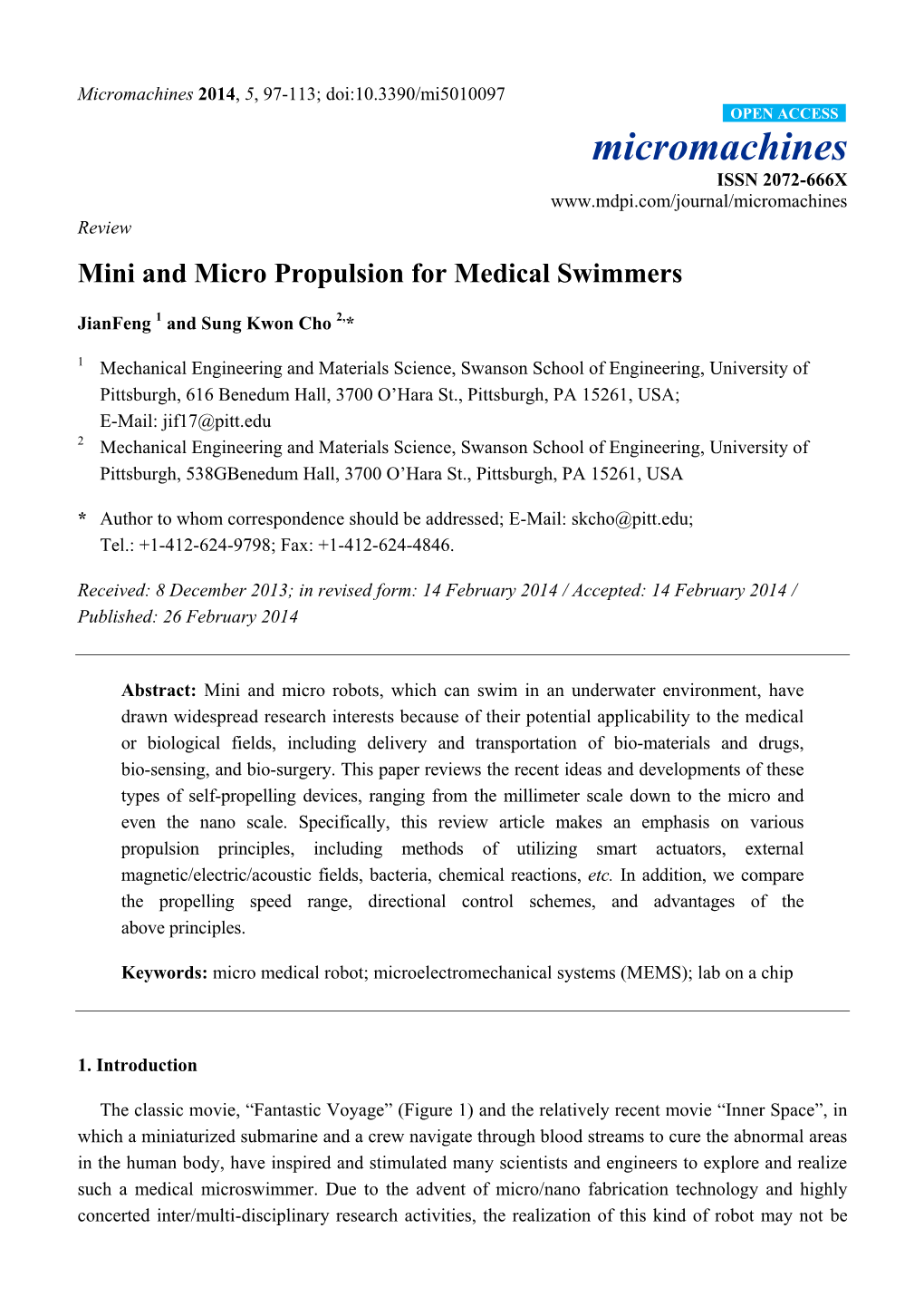
Load more
Recommended publications
-
Review of Advanced Medical Telerobots
applied sciences Review Review of Advanced Medical Telerobots Sarmad Mehrdad 1,†, Fei Liu 2,† , Minh Tu Pham 3 , Arnaud Lelevé 3,* and S. Farokh Atashzar 1,4,5 1 Department of Electrical and Computer Engineering, New York University (NYU), Brooklyn, NY 11201, USA; [email protected] (S.M.); [email protected] (S.F.A.) 2 Advanced Robotics and Controls Lab, University of San Diego, San Diego, CA 92110, USA; [email protected] 3 Ampère, INSA Lyon, CNRS (UMR5005), F69621 Villeurbanne, France; [email protected] 4 Department of Mechanical and Aerospace Engineering, New York University (NYU), Brooklyn, NY 11201, USA 5 NYU WIRELESS, Brooklyn, NY 11201, USA * Correspondence: [email protected]; Tel.: +33-0472-436035 † Mehrdad and Liu contributed equally to this work and share the first authorship. Abstract: The advent of telerobotic systems has revolutionized various aspects of the industry and human life. This technology is designed to augment human sensorimotor capabilities to extend them beyond natural competence. Classic examples are space and underwater applications when distance and access are the two major physical barriers to be combated with this technology. In modern examples, telerobotic systems have been used in several clinical applications, including teleoperated surgery and telerehabilitation. In this regard, there has been a significant amount of research and development due to the major benefits in terms of medical outcomes. Recently telerobotic systems are combined with advanced artificial intelligence modules to better share the agency with the operator and open new doors of medical automation. In this review paper, we have provided a comprehensive analysis of the literature considering various topologies of telerobotic systems in the medical domain while shedding light on different levels of autonomy for this technology, starting from direct control, going up to command-tracking autonomous telerobots. -
Control of Mobile Robot for Remote Medical Examination: Design Concepts and Users’ Feedback from Experimental Studies
Control of Mobile Robot for Remote Medical Examination: Design Concepts and Users’ Feedback from Experimental Studies Krzysztof Arent∗, Janusz Jakubiak∗, Michał Drwi˛ega∗, Mateusz Cholewinski´ ∗, Gerald Stollnbergery, Manuel Giulianiy, Manfred Tscheligiy, Dorota Szczesniak-Stanczykz, Marcin Janowskiz, Wojciech Brzozowskiz, Andrzej Wysokinski´ z ∗ Department of Cybernetics and Robotics, y Center for Human-Computer z Department of Cardiology Faculty of Electronics, Interaction, Medical University of Lublin, Wrocław University of Technology, University of Salzburg, ul. K. Jaczewskiego 8, Wybrzeze˙ Wyspianskiego´ 27, Sigmund-Haffner-Gasse 18, 20-143 Lublin, Poland 50-370 Wrocław, Poland 5020 Salzburg, Austria Email: [email protected] Email: [email protected] Email: [email protected] Abstract—In this article we discuss movement control of a ReMeDi medical mobile robot from the user perspective. The control is essentially limited to the level of operator actions where the operator is a member of a nursing staff. Two working modes are the base of considerations: long distance (LD) and short distance (SD) movement. In this context two robot control techniques are subject of study: manual with use of a gamepad and "point and click" on a map that is related to autonomous motion with use of an onboard navigation system. In the SD mode the user manually operates the robot, that is close to a Fig. 1. ReMeDi system overview [1] laying down patient on a settee. In the LD mode the mobile base moves autonomously in a space shared with people to the desired position. Two user studies were conducted. The results show that from the perspective of LD mode the autonomous acceptance from both patients and medical personnel. -
Efficient Design of Precision Medical Robotics
Efficient Design of Precision Medical Robotics by NEVAN CLANCY HANUMARA S.M. Mechanical Engineering Massachusetts Institute of Technology, 2006 B.S. Mechanical Engineering, B.A. French University of Rhode Island, 2004 Submitted to the Department of Mechanical Engineering in Partial Fulfillment of the Requirements for the Degree of Doctorate of Philosophy in Mechanical Engineering at the Massachusetts Institute of Technology September 2012 ©2012 Massachusetts Institute of Technology All Rights Reserved Author: Department of Mechanical Engineering 30 August 2012 Certified by: Alexander H. Slocum Pappalardo Professor of Mechanical Engineering Thesis Supervisor Accepted by: David E. Hardt Professor of Mechanical Engineering Graduate Officer Efficient Design of Precision Medical Robotics by NEVAN CLANCY HANUMARA Submitted to the M.I.T. Department of Mechanical Engineering on 30 August 2012 in Partial Fulfillment of the Requirements of the Degree of Doctorate of Philosophy in Mechanical Engineering Abstract Medical robotics is increasingly demonstrating the potential to improve patient care through more precise interventions. However, taking inspiration from industrial robotics has often resulted in large, sometimes cumbersome designs, which represent high capital and per procedure expenditures, as well as increased procedure times. This thesis proposes and demonstrates an alternative model and method for developing economical, appropriately scaled medical robots that improve care and efficiency, while moderating costs. Key to this approach -
MAPPING the DEVELOPMENT of AUTONOMY in WEAPON SYSTEMS Vincent Boulanin and Maaike Verbruggen
MAPPING THE DEVELOPMENT OF AUTONOMY IN WEAPON SYSTEMS vincent boulanin and maaike verbruggen MAPPING THE DEVELOPMENT OF AUTONOMY IN WEAPON SYSTEMS vincent boulanin and maaike verbruggen November 2017 STOCKHOLM INTERNATIONAL PEACE RESEARCH INSTITUTE SIPRI is an independent international institute dedicated to research into conflict, armaments, arms control and disarmament. Established in 1966, SIPRI provides data, analysis and recommendations, based on open sources, to policymakers, researchers, media and the interested public. The Governing Board is not responsible for the views expressed in the publications of the Institute. GOVERNING BOARD Ambassador Jan Eliasson, Chair (Sweden) Dr Dewi Fortuna Anwar (Indonesia) Dr Vladimir Baranovsky (Russia) Ambassador Lakhdar Brahimi (Algeria) Espen Barth Eide (Norway) Ambassador Wolfgang Ischinger (Germany) Dr Radha Kumar (India) The Director DIRECTOR Dan Smith (United Kingdom) Signalistgatan 9 SE-169 72 Solna, Sweden Telephone: +46 8 655 97 00 Email: [email protected] Internet: www.sipri.org © SIPRI 2017 Contents Acknowledgements v About the authors v Executive summary vii Abbreviations x 1. Introduction 1 I. Background and objective 1 II. Approach and methodology 1 III. Outline 2 Figure 1.1. A comprehensive approach to mapping the development of autonomy 2 in weapon systems 2. What are the technological foundations of autonomy? 5 I. Introduction 5 II. Searching for a definition: what is autonomy? 5 III. Unravelling the machinery 7 IV. Creating autonomy 12 V. Conclusions 18 Box 2.1. Existing definitions of autonomous weapon systems 8 Box 2.2. Machine-learning methods 16 Box 2.3. Deep learning 17 Figure 2.1. Anatomy of autonomy: reactive and deliberative systems 10 Figure 2.2. -
The Safety Issues of Medical Robotics
Reliability Engineering and System Safety 73 ,2001) 183±192 www.elsevier.com/locate/ress The safety issues of medical robotics Baowei Feia,1, Wan Sing Nga,*, Sunita Chauhana, Chee Keong Kwohb aSchool of Mechanical and Production Engineering, Nanyang Technological University, 50 Nanyang Avenue, Singapore 639798 bSchool of Computer Engineering, Nanyang Technological University, 50 Nanyang Avenue, Singapore 639798 Received 22 June 2000; accepted 10 April 2001 Abstract In this paper, we put forward a systematic method to analyze, control and evaluate the safety issues of medical robotics. We created a safety model that consists of three axes to analyze safety factors. Software and hardware are the two material axes. The third axis is the policy that controls all phases of design, production, testing and application of the robot system. The policy was de®ned as hazard identi®cation and safety insurance control ,HISIC) that includes seven principles: de®nitions and requirements, hazard identi®cation, safety insurance control, safety critical limits, monitoring and control, veri®cation and validation, system log and documentation. HISIC was implemented in the development of a robot for urological applications that was known as URObot. The URObot is a universal robot with different modules adaptable for 3D ultrasound image-guided interstitial laser coagulation, radiation seed implantation, laser resection, and electrical resection of the prostate. Safety was always the key issue in the building of the robot. The HISIC strategies were adopted for safety enhancement in mechanical, electrical and software design. The initial test on URObot showed that HISIC had the potential ability to improve the safety of the system. -
Medical Package for Patient Care
Patient care applications Robotics | Experts in Man and Machine STÄUBLI IN LIFE SCIENCE MORE THAN 30 YEARS OF EXPERIENCE Robots contributing Pioneer in robotics to human well being patient care For many years now, Stäubli Robotics Whenever accuracy has to be maintained Stäubli’s unique robot portfolio, decades of Stäubli PUMA 560 robot Stericlean range BHS Technologies Launch of dedicated range Surgery assistance has been on a mission to develop robots alongside factors such as particle industry experience and strong customer Brain biopsy assistance Medtech (needle placement, scanner for medical and pharma Zimmer Biomet (precision vision for life science applications. Stäubli emissions, easy-to-clean surfaces and focus make it a valued partner for renowned support) applications Knee surgery positioning) robots are setting the benchmark for availability, Stäubli has long led the way. life science companies around the globe. 1985 2008 2018 2020 hygiene and safety. 1998 2013 2019 Quantum Surgical Partnership for liver Ortho Maquet Medtech Keranova surgery Hip and knee surgery Spine and brain Cataract operation surgery Life Science H Brain intervention support: Epilepsy, brain tumors, Laboratory research, Pharmaceuticals Manufacturing of Hospital Patient care behavior disorder, drug discovery and biotechnology medical devices automation Parkinson desease and diagnostics Hair transplantation Cataract and eye surgery Hearing implants Dental corrections Shoulder arthroscopy Liver cancer surgery Diagnosis Spine surgery Hip replacement Knee arthroplasty Robotics | Stäubli's offer for patient care applications 3 DESIGNED FOR PATIENT CARE DESIGNED FOR PATIENT CARE Medical package Your partner for safer intervention Stäubli has developed a unique offer to As in our industrial solutions, the medical Stäubli currently operates in 29 countries, meet a number of constraints present in range ensures consistent performance and with agents in 50 countries to provide patient care. -
Advanced Robotic Systems
INTERNATIONAL JOURNAL OF ADVANCED ROBOTIC SYSTEMS Books of Abstracts| Volume 11 | 2014 | ISSN 1729-8806 International Journal of Advanced Robotic Systems Book of Abstracts Volume 11, 2014 This Book of Abstracts covers the titles, authors, abstracts and keywords of the articles published within Volume 11 of the International Journal of Advanced Robotic Systems. For each of the published articles in 2014 readers can find the link which will lead them to the designated web page and a full-text article available for download. ISSN 1729-8806 www.intechopen.com A New Profile Shape Matching Stereovision Algorithm for Real-time Human Pose Table of Contents and Hand Gesture Recognition Dong Zhang, Dah-Jye Lee and Yung-Ping Chang 17 Modelling, Design and Robust Control of a Remotely Operated Underwater Vehicle Luis Govinda García-Valdovinos, Tomás Salgado-Jiménez, A Simulation Environment for Bio-inspired Heterogeneous Chained Modular Robots Manuel Bandala-Sánchez, Luciano Nava-Balanzar, Rodrigo Hernández-Alvarado Alberto Brunete, Miguel Hernando and Ernesto Gambao 18 and José Antonio Cruz-Ledesma 10 A Novel Robust Scene Change Detection Algorithm for Autonomous Robots Stitching Images with Arbitrary Lens Distortions Using Mixtures of Gaussians Myung-Ho Ju and Hang-Bong Kang 10 Luis J. Manso, Pedro Núñez, Sidnei da Silva and Paulo Drews-Jr 18 An Adaptive Neural Network Learning-Based Solution for the Inverse Kinematics Online Joint Trajectory Generation of Human-like Biped Walking of Humanoid Fingers Jong-Wook Kim 19 Byoung-Ho Kim 11 An Underactuated Multi-finger Grasping Device An Efficient Ceiling-view SLAM Using Relational Constraints Between Landmarks Cesare Rossi and Sergio Savino 19 Hyukdoo Choi, Ryunseok Kim and Euntai Kim 11 Quantile Acoustic Vectors vs. -
High Performance Medical Robot Requirements and Accuracy Analysis C
High Performance Medical Robot Requirements and Accuracy Analysis C. Mavroidis1, J. Flanz2, S. Dubowsky 3, P. Drouet4 and Michael Goitein2 1 Department of Mechanical and Aerospace Engineering, Rutgers University 98 Brett Road, Room B-217, Piscataway, NJ 08854, USA Tel 732-445-0732, Fax 732-445-3124, email [email protected] 2 Northeast Proton Therapy Center, Massachusetts General Hospital Boston, MA 02114 USA, Tel 617-724-9528, Fax 617-724-9532 3 Massachusetts Institute of Technology, Department of Mechanical Engineering Cambridge, MA 02139, USA, Tel 617-253-2144, Fax 617-258-7881 4 Laboratoire de Mécanique des Solides, Université de Poitiers Bât. SP2MI, 86960 Futuroscope, FRANCE, Tel 05.49.49.65.00 Abstract The treatment of disease using particle beams requires highly accurate patient positioning. Patients must be well immobilized and precisely aligned with the treatment beam to take full advantage of the dose localization potential. Robots can be used as high accuracy patient positioning systems. In this paper, the first such implementation using robotics techniques for patient positioning will be discussed. This robot is being developed for the Northeast Proton Therapy Center at the Massa- chusetts General Hospital. The unique requirements and design characteristics of the patient posi- tioning system are presented. Of special interest is the system's patient positioning accuracy. A systematic methodology to perform the error analysis of serial link manipulators and its application to the PPS is described. Experimental measurements that verified the validity of the method are shown. 1 INTRODUCTION The application of robotic technology in clinical medicine has recently been a very active research area [7]. -
Gwell Medical Solution Brief
Solution Brief Medical and Life Sciences Gwell Medical (mRobot) Optimizes Hospital Logistics Robotic Solution Based on Intel® Architecture The Noah robot is an excellent substitute for human labor. A logistics worker can usually handle up to 30 kg at a time, while the Noah robot can carry up to 300 kg. As labor costs increase and hospitals require more stringent logistics management, a traditional human labor approach will make it hard to meet Authors: these challenges. In the future, hospitals will have trends similar to factories: Zhen (Fiona) Zhao more robots will gradually assist with these changing demands. Deep learning technical consulting engineer, Intel Corporation – Wei Lü Cofounder and CTO Lei Zhang Software algorithm engineer, Gwell Medical Gwell Medical Technology Co., Ltd. Xuesong Shi Gwell’s Noah hospital robot uses Intel® technologies to perform Research scientist, Intel Labs China, many human tasks Intel Corporation Hospital business operations have significant logistics demands for the distribution of Dmitry Kurtaev medications, consumables, food and beverages, and for waste removal. Technologies including Deep learning software engineer, robotics, computer vision, artificial intelligence (AI), and deep learning (DL) are automating Intel Corporation logistics operations in hospitals to help improve efficiency and streamline human workloads. Gan Zhang Navigation control development manager, To address these rapidly evolving needs, Shanghai Gwell Medical (formerly known as mRobot) Gwell Medical Technology Co., Ltd. developed the Noah hospital logistics robot based on an Intel® Core™ processor, Intel® Movidius™ vision processing unit (VPU), and Intel® Distribution of OpenVINO™ Hengkang Liang toolkit. The Noah robot incorporates cutting-edge technologies such as autonomous Hardware engineering director, Gwell Medical navigation, elevator access, loading/unloading, and multirobot scheduling. -
Biomedical Applications of Untethered Mobile Milli/Microrobots This Paper Reviews the Current Advances in Biomedical Untethered Mobile Millirobots and Microrobots
INVITED PAPER Biomedical Applications of Untethered Mobile Milli/Microrobots This paper reviews the current advances in biomedical untethered mobile millirobots and microrobots. By Metin Sitti, Fellow IEEE, Hakan Ceylan, Wenqi Hu, Student Member IEEE, Joshua Giltinan, Student Member IEEE, Mehmet Turan, Sehyuk Yim, Student Member IEEE,andEricDiller,Member IEEE ABSTRACT | Untethered robots miniaturized to the length I. INTRODUCTION scale of millimeter and below attract growing attention for the One of the highest potential scientific and societal impacts prospect of transforming many aspects of health care and of small-scale (millimeter and submillimeter size) unteth- bioengineering. As the robot size goes down to the order of a ered mobile robots would be their healthcare and bioen- single cell, previously inaccessible body sites would become gineering applications. As an alternative to existing tethered available for high-resolution in situ and in vivo manipulations. medical devices such as flexible endoscopes and catheters, This unprecedented direct access would enable an extensive mobile medical milli/microrobots could access complex and range of minimally invasive medical operations. Here, we pro- small regions of the human body such as gastrointestinal vide a comprehensive review of the current advances in biome (GI), brain, spinal cord, blood capillaries, and inside the eye dical untethered mobile milli/microrobots. We put a special while being minimally invasive and could even enable emphasis on the potential impacts of biomedical microrobots access to unprecedented submillimeter size regions inside in the near future. Finally, we discuss the existing challenges the human body, which have not been possible to access and emerging concepts associated with designing such a currently with any medical device technology [1], [2]. -
Bringing Surgical Insight to Medical Robot Design
ADVERTISEMENT FEATURE ADVERTISEMENT FEATURE A research team at the Third Xiangya Hospital of Central South University advised on the medical robot design. Bringing surgical insight to medical robot design obot-assisted surgery is safety, a clearer view through becoming increasingly the endoscope to allow for R common for its greater precise operation, increased stability, precision, and fl exibility user comfort to reduce fatigue, for complex procedures. From and shorter time under minimally invasive endoscopic anaesthesia. surgery, to complicated heart or Clinical researchers from brain surgeries, robotic systems the Third Xiangya Hospital of are widely used, with the growing Central South University have demand for minimally invasive advised on the initial design procedures that are less painful with their clinical experience. and safer for patients. Their rich data helped inform In China, the surgical robot the establishment of evaluation DEVELOPMENT OF MICRO HAND S industry is still in its infancy. criteria for product safety In the ‘Medium-to-Long-Term and e ectiveness. In the Plan for the Development of development of the prototype Science and Technology’ issued machine, researchers from the 1 by the government in 2006, hospital also performed in vitro the development of intelligent and animal experiments, as well robots was, for the fi rst time, as clinical trials to guarantee earmarked as being of national evidence-based design. strategic importance, and listed After more than 10 years as a cutting-edge technology of research, design, evaluation for boosting advanced tests, and retrospective and manufacturing. prospective clinical studies, In line with this, in 2008, the prototype was updated and when the da Vinci Surgical improved. -
Analysis and Synthesis of Parallel Robots for Medical Applications
Analysis and Synthesis of Parallel Robots for Medical Applications Nabil Simaan Analysis and Synthesis of Parallel Robots for Medical Applications RESEARCH THESIS SUBMITTED IN PARTIAL FULFILLMENT OF THE REQUIREMENTS FOR THE DEGREE OF MASTER OF SCIENCE IN MECHANICAL ENGINEERING by Nabil Simaan SUBMITTED TO THE SENATE OF THE TECHNION – ISRAEL INSTITUTE OF TECHNOLOGY HAIFA July 99 אב תשנ"ט This research was conducted under the supervision of Prof. Moshe Shoham in the Faculty of Mechanical Engineering , Technion – Israel Institute of Technology. Acknowledgments I am grateful for the dedicated supervision of Prof. Moshe Shoham. I spent about three busy years working with him in which I learned to appreciate his perseverance in research. I appreciate the time he spent with me on realizing this work both theoretically and especially practically in the stage of developing the prototype. I thank Mrs. Hanna Maller for her patience in answering all my endless questions regarding the bureaucratic procedures during my study period. I would like to thank the technical staff of the workshop in the robotics and manufacturing building of the Mechanical Engineering faculty for their work in preparing the prototype. Special thanks are dedicated to Yossi Barzily who expressed in stages of manufacturing his critical thoughts about my design and made me think about design issues repeatedly. I appreciate the work done by Avi Suliman who prepared the electrical system. Without his professional work, the electrical setup for the prototype would have been still missing. The generous financial support of the Technion, Mitchell-Sorf Foundation, and Marco and Louise Mitrani Memorial Fellowship is gratefully acknowledged.