Stratigraphy, Sedimentation and Age of The
Total Page:16
File Type:pdf, Size:1020Kb
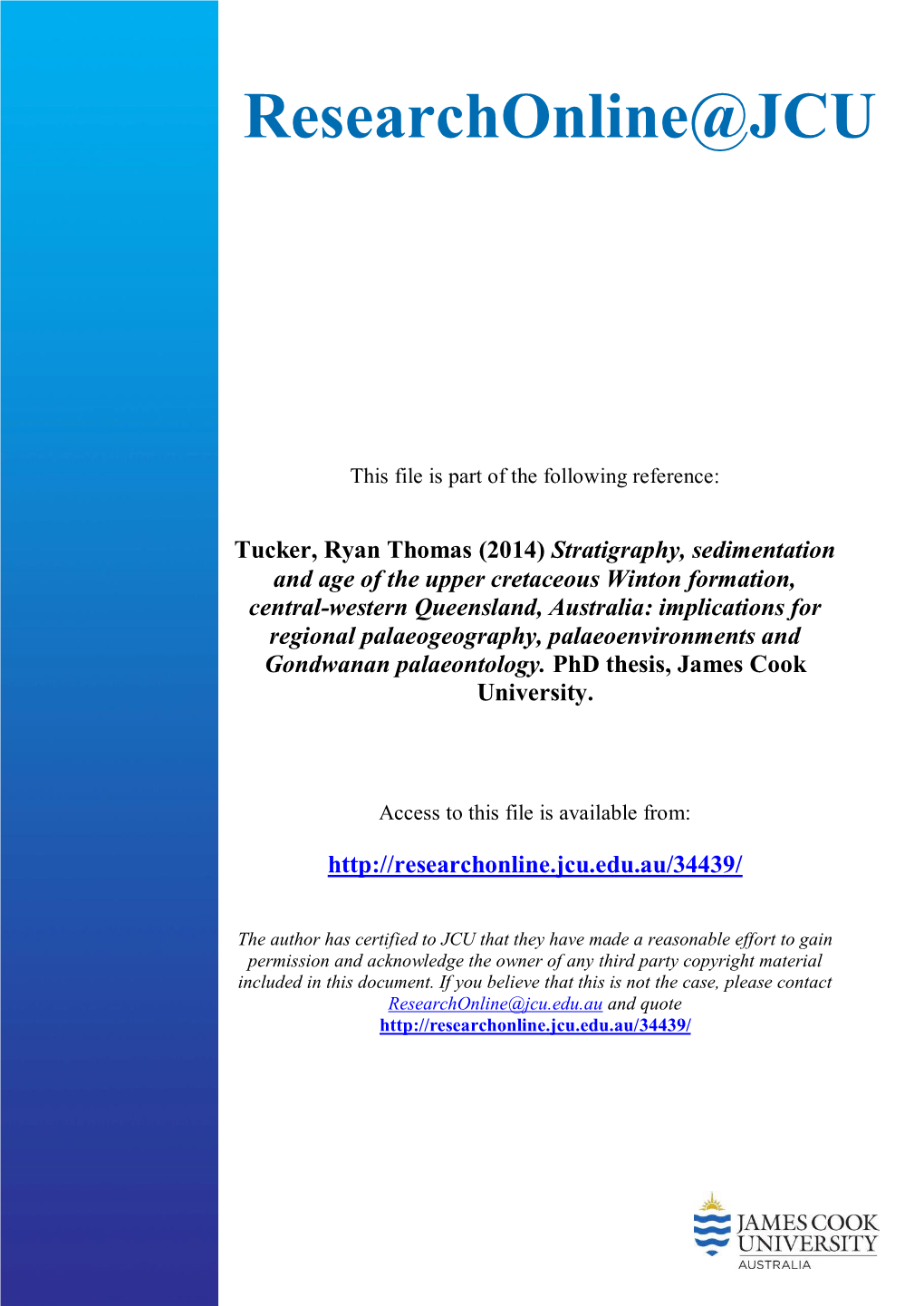
Load more
Recommended publications
-
Regolith-Landform Evolution on Cape York Peninsula
REGOLITH-LANDFORM EVOLUTION ON CAPE YORK PENINSULA REGOLITH-LANDFORM EVOLUTION ON CAPE YORK PENINSULA: IMPLICATIONS FOR MINERAL EXPLORATION C F PAIN 1, J R WILFORD 1 AND J C DOHRENWEND2 1 Cooperative Research Centre for Landscape Evolution and Mineral Exploration, c/- Australian Geological Survey Organisation, PO Box 378, Canberra ACT 2601, Australia 25755 East River Road #3207, Tucson, AZ 85750, USA A number of landform features on Cape York Peninsula (CYP) contain important clues for the development of landforms and regolith in the area The Great Escarpment, up to 200 m high, separates old landforms and regolith on the westem side from younger landforms and regolith on the eastem side West of the Great Escarpment there are smaller less continuous scarps which also form important boundaries between different regolith types There is clear eVidence for superimposed drainage, river capture and reversal, and inversion of relief on CYP Formation of the Carpentaria and Laura Basins during Middle to Late Jurassic and Early Cretaceous time covered the Palaeozoic and Proterozoic rocks of the Coen Inlier with coarse terrestrial to fine marine sediment The uplift and emergence of these basin sediments in the Late Cretaceous marks the beginning of the latest stage of landform and regolith evolution on CYP The sediments probably covered most, if not all, of the Coen Inlier, and post-Mesozoic erosion subsequently uncovered the basement to form the Inlier Substantial erosion and surface lowering in the area followed emergence at the end of the Cretaceous, -
John Hillier HYDROGEOLOGY Great Artesian Basin /Galilee Basin
HYDROGEOLOGY Great Artesian Basin /Galilee Basin Presented by John Hillier Acknowledgement Part of this presentation includes some of the findings of a report commissioned by members of the Galilee Basin Operators Forum (GBOF). The report was compiled by RPS Australia East Pty Ltd. Some Maps and Figures form their report have been used in this presentation. Location of GAB & Galilee Basins The Galilee Basin partly underlies the Great Artesian Basin Both were deposited on a very uneven surface STRATIGRAPHY AGE BASIN FORMATION ROLLING DOWNS GROUP (e.g. Winton Formation, Wallumbilla Formation, etc) CRETACEOUS CADNA-OWIE FORMATION (66 – 145 Myr) EROMANGA HOORAY SANDSTONE (GREAT ARTESIAN WESTBOURNE FORMATION BASIN) ADORI SANDSTONE JURASSIC BIRKHEAD FORMATION (145 – 200 Myr) HUTTON SANDSTONE GALILEE MOOLAYEMBER FORMATION TRIASSIC (GREAT ARTESIAN CLEMATIS SANDSTONE (200 – 253 Myr) BASIN) REWAN FORMATION (includes Dundas Beds) BETTS CREEK BEDS (Includes Bandanna PERMIAN Formation and Colinlea Sandstone) GALILEE (253 – 299 Myr) JOE JOE FORMATION (Includes Aramac Coal Measures) GAB - Surface Geology Eastern Area Outcrop Galilee Basin Coal Coal is mainly in the Colinlea Sandstone and the overlying Bandana Formation Near outcrop, where it is to be mined for coal, the coal seams range up to 8m in thickness Seam thicknesses vary considerable throughout the Basin How does the GAB operate •Water is stored in sandstone aquifers •Recharge is from rainfall that enters the aquifers where they outcrop at the surface •Groundwater movement in the GAB -
From the Early Cretaceous Wonthaggi Formation (Strzelecki Group)
Journal of Paleontology, 93(3), 2019, p. 543–584 Copyright © 2019, The Paleontological Society. This is an Open Access article, distributed under the terms of the Creative Commons Attribution licence (http://creativecommons.org/ licenses/by/4.0/), which permits unrestricted re-use, distribution, and reproduction in any medium, provided the original work is properly cited. 0022-3360/19/1937-2337 doi: 10.1017/jpa.2018.95 New small-bodied ornithopods (Dinosauria, Neornithischia) from the Early Cretaceous Wonthaggi Formation (Strzelecki Group) of the Australian-Antarctic rift system, with revision of Qantassaurus intrepidus Rich and Vickers-Rich, 1999 Matthew C. Herne,1,2 Jay P. Nair,2 Alistair R. Evans,3 and Alan M. Tait4 1School of Environmental and Rural Science, University of New England, Armidale 2351, New South Wales, Australia <ornithomatt@ gmail.com> 2School of Biological Sciences, The University of Queensland, Brisbane, Queensland 4072, Australia <[email protected]> 3School of Biological Sciences, Monash University, Melbourne, Victoria 3800, Australia <[email protected]> 4School of Earth, Atmosphere & Environment, Monash University, Melbourne, Victoria 3800, Australia <[email protected]> Abstract.—The Flat Rocks locality in the Wonthaggi Formation (Strzelecki Group) of the Gippsland Basin, southeastern Australia, hosts fossils of a late Barremian vertebrate fauna that inhabited the ancient rift between Australia and Antarc- tica. Known from its dentary, Qantassaurus intrepidus Rich and Vickers-Rich, 1999 has been the only dinosaur named from this locality. However, the plethora of vertebrate fossils collected from Flat Rocks suggests that further dinosaurs await discovery. From this locality, we name a new small-bodied ornithopod, Galleonosaurus dorisae n. -
Tartu, 26.-27. September 2003 Elga Mark-Kurik Ja Jйri Nemliher Tallinna
Eesti geoloogia uue sajandi kiinnisel 38 Tartu, 26.-27. september 2003 VEEL KORD КESK-DEVONI АВА V А КIНТIDEST Elga Mark-Kurik ja Jйri Nemliher Tallinna Tehnikatilikooli Geoloogia Instituut, Estonia pst. 7, 10143 Tallinn ([email protected]; [email protected]) Abava kihid on kauaaegse asendi tottu Kesk- ja Ulem-Devoni piiril pohjustanud rohkem vaidlusi kui mбni teine stratigraafiline tiksus Baltikumi Нibiloigetes. Selle asendiga seoses on neid kihte kasitletud kord iseseisva tiksusena (Liepins 1960, Mark-Kurik 1991), marksa sagedamini aga lasuva, Gauja lademe osana (Kurss et al. 1981, Mark-Kurik 1981) vбi lamava, Burtnieki lademe osana (Kleesment 1995, Kleesment & Mark-Kurik 1997, Vingisaar 1997). Abava kihtide kui Uksuse liitmist selle vбi teise kбrgemat jarku Uksusega on pбhjustanud antud Uksuse piiride, eriti Ulemise piiri ebamaarasus ning halb jalgitavus geoloogilisel kaш·distamisel (Kurik et al. 1989). Viimatimainitud asjaolu tottu loobus Kurss hiljem ( 1992) Abava kui iseseisva stratigraafilise tiksuse kasutamisest. Siiski ei saa mainimata jatta Abava kihtidele spetsiifilist kalafaunat, samuti selle tiksikelementide.laia levikut Ida Euroopa platvormil ning naaberaladel ja kaugemalgi ning nimetatud kihtide tllhtsust regioonidevahelise korrelatsiooni aspektist (Mark-Kurik 1991, Ahlberg et al. 1999, Mark-Kurik et al. 1999). Eestis kuuluvad Abava kihid Givet' ladejargu Burtnieki lademesse, moodustades selle kolmanda ehk Ulemise kihikompleksi. Кihtide paksus on 15,1-32 m. Need koosnevad heledavarvilisest peeneteralisest pбimjaskihilisest liivakivist, ka peeneteralisest hallist, rohekas- voi lillakashallist aleuroliidist. Aleuroliiti on antud Uksuses enam kui 25 %. Samuti esineb selles halli voi punakaspruuni savi. Kagu-Eesti puurstidamikes on Abava tasemelt leitud dolokivi ning domeriiti. Mineraloogiliselt on meie Vбhandu jбе aarsed paljandid ning Latis paiknev Lejeji paljand sarnased (Kleesment 1995). Eelpool mainitud paljand (stratottiiip) asub Kuramaal Venta jбgikonnas Abava jбе vasakul kaldal (suudmest 4 km Ulesvoolu) Lejeji talu vastas. -
Unconventional Gas Production
Engineering Energy: Unconventional Gas Production A study of shale gas in Australia. FINAL REPORT PROJECT AUSTRALIAN ACADEMY OF THE HUMANITIES AUSTRALIAN ACADEMY OF SCIENCE ACADEMY OF THE SOCIAL SCIENCES IN AUSTRALIA AUSTRALIAN ACADEMY OF TECHNOLOGICAL SCIENCES AND ENGINEERING SECURING EXPERT AUSTRALIA’S WORKING FUTURE GROUP – PROJECT 6 A three-year research Professor Peter Cook CBE, FTSE (Chair) program funded by the Dr Vaughan Beck FTSE (Deputy Chair) Australian Research Professor David Brereton Council and conducted Professor Robert Clark AO, FAA, FRSN Dr Brian Fisher AO, PSM, FASSA by the four Learned Professor Sandra Kentish Academies through Mr John Toomey FTSE the Australian Council Dr John Williams FTSE of Learned Academies for PMSEIC, through AUTHORS the Office of the Chief Professor Peter Cook CBE, FTSE Scientist. Securing Dr Vaughan Beck FTSE Australia’s Future delivers Professor David Brereton research-based evidence Professor Robert Clark AO, FAA, FRSN and findings to support Dr Brian Fisher AO, PSM, FASSA policy development in Professor Sandra Kentish areas of importance to Mr John Toomey FTSE Australia’s future. Dr John Williams FTSE © Australian Council of Learned Academies (ACOLA) ISBN 978 0 9875798 1 2 This work is copyright. Apart from any use permitted under the Copyright Act 1968, no part of it may be reproduced by any process without written permission from the publisher. Requests and inquiries concerning reproduction rights should be directed to the publisher. DATE OF PUBLICATION May 2013 PUBLISHER Australian Council of Learned Academies Level 1, 1 Bowen Crescent Melbourne Victoria 3004 Australia Telephone: +61 (0)3 98640923 www.acola.org.au SUGGESTED CITATION Cook, P, Beck, V, Brereton, D, Clark, R, Fisher, B, Kentish, S, Toomey, J and Williams, J (2013). -
Poropat Et Al 2017 Reappraisal Of
Alcheringa For Peer Review Only Reappraisal of Austro saurus mckillopi Longman, 1933 from the Allaru Mudstone of Queensland, Australia’s first named Cretaceous sauropod dinosaur Journal: Alcheringa Manuscript ID TALC-2017-0017.R1 Manuscript Type: Standard Research Article Date Submitted by the Author: n/a Complete List of Authors: Poropat, Stephen; Swinburne University of Technology, Department of Chemistry and Biotechnology; Australian Age of Dinosaurs Natural History Museum Nair, Jay; University of Queensland, Biological Sciences Syme, Caitlin; University of Queensland, Biological Sciences Mannion, Philip D.; Imperial College London, Earth Science and Engineering Upchurch, Paul; University College London, Earth Sciences, Hocknull, Scott; Queensland Museum, Geosciences Cook, Alex; Queensland Museum, Palaeontology & Geology Tischler, Travis; Australian Age of Dinosaurs Natural History Museum Holland, Timothy; Kronosaurus Korner <i>Austrosaurus</i>, Dinosauria, Sauropoda, Titanosauriformes, Keywords: Australia, Cretaceous, Gondwana URL: http://mc.manuscriptcentral.com/talc E-mail: [email protected] Page 1 of 126 Alcheringa 1 2 3 4 5 6 7 1 8 9 1 Reappraisal of Austrosaurus mckillopi Longman, 1933 from the 10 11 12 2 Allaru Mudstone of Queensland, Australia’s first named 13 14 For Peer Review Only 15 3 Cretaceous sauropod dinosaur 16 17 18 4 19 20 5 STEPHEN F. POROPAT, JAY P. NAIR, CAITLIN E. SYME, PHILIP D. MANNION, 21 22 6 PAUL UPCHURCH, SCOTT A. HOCKNULL, ALEX G. COOK, TRAVIS R. TISCHLER 23 24 7 and TIMOTHY HOLLAND 25 26 27 8 28 29 9 POROPAT , S. F., NAIR , J. P., SYME , C. E., MANNION , P. D., UPCHURCH , P., HOCKNULL , S. A., 30 31 10 COOK , A. G., TISCHLER , T.R. -
Epm 16511 Brodies Lookout
Investigator Resources Limited: Surrender and Final Report EPM 16511 “Brodies Lookout” Investigator Resources Limited Suite 48, Level 3, Benson House 2 Benson Street, Toowong, Qld 4066 Tel: +61 (0)7 3870 0357 Fax: +61 (0)7 3876 0351 Email: [email protected] web: www.investres.com.au EPM 16511 BRODIES LOOKOUT SURRENDER AND FINAL REPORT for period 27 April 2009 to 26 April 2011 D G Jones and B R Willott Investigator Resources Limited 6 September 2011 Page | 1 Investigator Resources Limited: Surrender and Final Report EPM 16511 “Brodies Lookout” 2 TABLE OF CONTENTS 3. SUMMARY ...................................................................................................................................................... 4 4. INTRODUCTION ............................................................................................................................................ 5 4.1. TENURE ....................................................................................................................................................... 5 4.2. LOCATION ................................................................................................................................................... 6 4.3. LOCAL ACCESS ........................................................................................................................................... 7 4.4. EXPLORATION RATIONALE ......................................................................................................................... 8 4.5. PROGRAM UNDERTAKEN -
Geology and Mineral Resources of the Northern Territory
Geology and mineral resources of the Northern Territory Ahmad M and Munson TJ (compilers) Northern Territory Geological Survey Special Publication 5 Chapter 41: Eromanga Basin BIBLIOGRAPHIC REFERENCE: Munson TJ, 2013. Chapter 41: Eromanga Basin: in Ahmad M and Munson TJ (compilers). ‘Geology and mineral resources of the Northern Territory’. Northern Territory Geological Survey, Special Publication 5. Disclaimer While all care has been taken to ensure that information contained in this publication is true and correct at the time of publication, changes in circumstances after the time of publication may impact on the accuracy of its information. The Northern Territory of Australia gives no warranty or assurance, and makes no representation as to the accuracy of any information or advice contained in this publication, or that it is suitable for your intended use. You should not rely upon information in this publication for the purpose of making any serious business or investment decisions without obtaining independent and/or professional advice in relation to your particular situation. The Northern Territory of Australia disclaims any liability or responsibility or duty of care towards any person for loss or damage caused by any use of, or reliance on the information contained in this publication. Eromanga Basin Current as of May 2012 Chapter 41: EROMANGA BASIN TJ Munson INTRODUCTION geology and regolith). However, the southwestern margins of the basin are exposed in SA and the northeastern part of The Cambrian±"Devonian Warburton Basin, the basin in central Qld is also exposed and has been eroding Carboniferous±Triassic 3edirNa Basin and Jurassic± since the Late Cretaceous. -
EGT Aastaraamat 2020 ENG.Indd
YEARBOOK GEOLOGICAL SURVEY OF ESTONIA F. R. Kreutzwaldi 5 44314 Rakvere Telephone: (+372) 630 2333 E-mail: [email protected] ISSN 2733-3337 © Eesti Geoloogiateenistus 2021 2 Foreword . 3 About GSE . 5 Cooperation . 6 Human resource development . 9 Fieldwork areas 2020 . 12 GEOLOGICAL MAPPING AND GEOLOGICAL DATA Coring – a major milestone in subsurface investigations in Estonia . 13 Coring projects for geological investigations at the GSE in 2020 . 15 Distribution, extraction, and exploitation of construction minerals in Pärnu county . 17 Mineral resources, geophysical anomalies, and Kärdla Crater in Hiiumaa . .22 Geological mapping in Pärnu County . .26 Opening year of the digital Geological Archive . .29 HYDROGEOLOGY AND ENVIRONMENTAL GEOLOGY Status of Estonian groundwater bodies in 2014–2019 . 31 Salinisation of groundwater in Ida-Viru County . .35 Groundwater survey of Kukruse waste rock heap . .37 The quality of groundwater and surface water in areas with a high proportion of agricultural land . .39 Transient 3D modelling of 18O concentrations with the MODFLOW-2005 and MT3DMS codes in a regional-scale aquifer system: an example from the Estonian Artesian Basin . .42 Radon research in insuffi ciently studied municipalities: Keila and Võru towns, Rõuge, Setomaa, Võru, and Ruhnu rural municipalities . .46 GroundEco – joint management of groundwater dependent ecosystems in transboundary Gauja–Koiva river basin . .50 MARINE GEOLOGY Coastal monitoring in 2019-2020 . .53 Geophysical surveys of fairways . .56 Environmental status of seabed sediments in the Baltic Sea . .58 The strait of Suur väin between the Estonian mainland and the Muhu Island overlies a complex bedrock valley . 60 Foreword 2020 has been an unusual year that none of us is likely to soon forget. -
Land Zones of Queensland
P.R. Wilson and P.M. Taylor§, Queensland Herbarium, Department of Science, Information Technology, Innovation and the Arts. © The State of Queensland (Department of Science, Information Technology, Innovation and the Arts) 2012. Copyright inquiries should be addressed to <[email protected]> or the Department of Science, Information Technology, Innovation and the Arts, 111 George Street, Brisbane QLD 4000. Disclaimer This document has been prepared with all due diligence and care, based on the best available information at the time of publication. The department holds no responsibility for any errors or omissions within this document. Any decisions made by other parties based on this document are solely the responsibility of those parties. Information contained in this document is from a number of sources and, as such, does not necessarily represent government or departmental policy. If you need to access this document in a language other than English, please call the Translating and Interpreting Service (TIS National) on 131 450 and ask them to telephone Library Services on +61 7 3224 8412. This publication can be made available in an alternative format (e.g. large print or audiotape) on request for people with vision impairment; phone +61 7 3224 8412 or email <[email protected]>. ISBN: 978-1-920928-21-6 Citation This work may be cited as: Wilson, P.R. and Taylor, P.M. (2012) Land Zones of Queensland. Queensland Herbarium, Queensland Department of Science, Information Technology, Innovation and the Arts, Brisbane. 79 pp. Front Cover: Design by Will Smith Images – clockwise from top left: ancient sandstone formation in the Lawn Hill area of the North West Highlands bioregion – land zone 10 (D. -
Context Statement for the Central West Subregion, PDF, 14.08 MB
1 Context statement for the Central West subregion Product 1.1 for the Northern Inland Catchments Bioregional Assessment 29 August 2014 A scientific collaboration between the Department of the Environment, Bureau of Meteorology, CSIRO and Geoscience Australia The Bioregional Assessment Programme The Bioregional Assessment Programme is a transparent and accessible programme of baseline assessments that increase the available science for decision making associated with coal seam gas and large coal mines. A bioregional assessment is a scientific analysis of the ecology, hydrology, geology and hydrogeology of a bioregion with explicit assessment of the potential direct, indirect and cumulative impacts of coal seam gas and large coal mining development on water resources. This Programme draws on the best available scientific information and knowledge from many sources, including government, industry and regional communities, to produce bioregional assessments that are independent, scientifically robust, and relevant and meaningful at a regional scale. The Programme is funded by the Australian Government Department of the Environment. The Department of the Environment, Bureau of Meteorology, CSIRO and Geoscience Australia are collaborating to undertake bioregional assessments. For more information, visit <http://www.bioregionalassessments.gov.au>. Department of the Environment The Office of Water Science, within the Australian Government Department of the Environment, is strengthening the regulation of coal seam gas and large coal mining development by ensuring that future decisions are informed by substantially improved science and independent expert advice about the potential water related impacts of those developments. For more information, visit <http://www.environment.gov.au/coal-seam-gas-mining/>. Bureau of Meteorology The Bureau of Meteorology is Australia’s national weather, climate and water agency. -
Systematics and Biostratigraphy of Australian Early Cretaceous
This file is part of the following reference: Williamson, Toni (2006) Systematics and biostratigraphy of Australian early cretaceous belemnites with contributions to the timescale and palaeoenvironmental assessment of the early Australian early cretaceous system derived from stable isotope proxies. PhD thesis, James Cook University. Access to this file is available from: http://eprints.jcu.edu.au/4906 " SECTION!A! ! ! ! ! ! ! Albian"and"Cenomanian"belemnites"of"the"Family"Dimitobelidae" from"Australia" Section!A.!!Albian!and!Cenomanian!Dimitobelidae!from!Australia! Section!A.! Albian!and!Cenomanian!belemnites!of!the!Family!Dimitobelidae! from!Australia" " A.!1!Abstract! Representatives" of" the" Family" Dimitobelidae" (Mollusca:Cephalopoda)! are" abundantly" represented"in"Australian"Early"Cretaceous"shallow"marine"strata,"especially"those"related"to" the"epeiric"sedimentary"record"of"the"Great"Artesian"Basin.""The"taxonomy,"biostratigraphy"and" distribution" of" Albian" and" Cenomanian" Australian" representatives" of" the" family" are" revised," utilising" all" known" collections." " The" genus" Dimitobelus" Whitehouse" (early" Albian" !" mid" Cenomanian)"occurs"in"both"the"Great"Artesian"Basin"of"eastern"Australia"and"the"Carnarvon" Basin" of" Western" Australia." This" genus" embraces" six" named" species:" " D.! diptychus" (McCoy)" (earliest"Albian"!"mid"Cenomanian),"D.!stimulus" Whitehouse"(Albian),"D.!dayi" Doyle"(earliest" Albian" –" early" late" Albian)," D.! liversidgei" Etheridge" (late" Albian)," D.! plautus" sp." nov" (early"