Osmotic and Activity Coefficients of the {Xzncl2 + (1 - X)Znso4}(Aq) System at 298.15 K
Total Page:16
File Type:pdf, Size:1020Kb
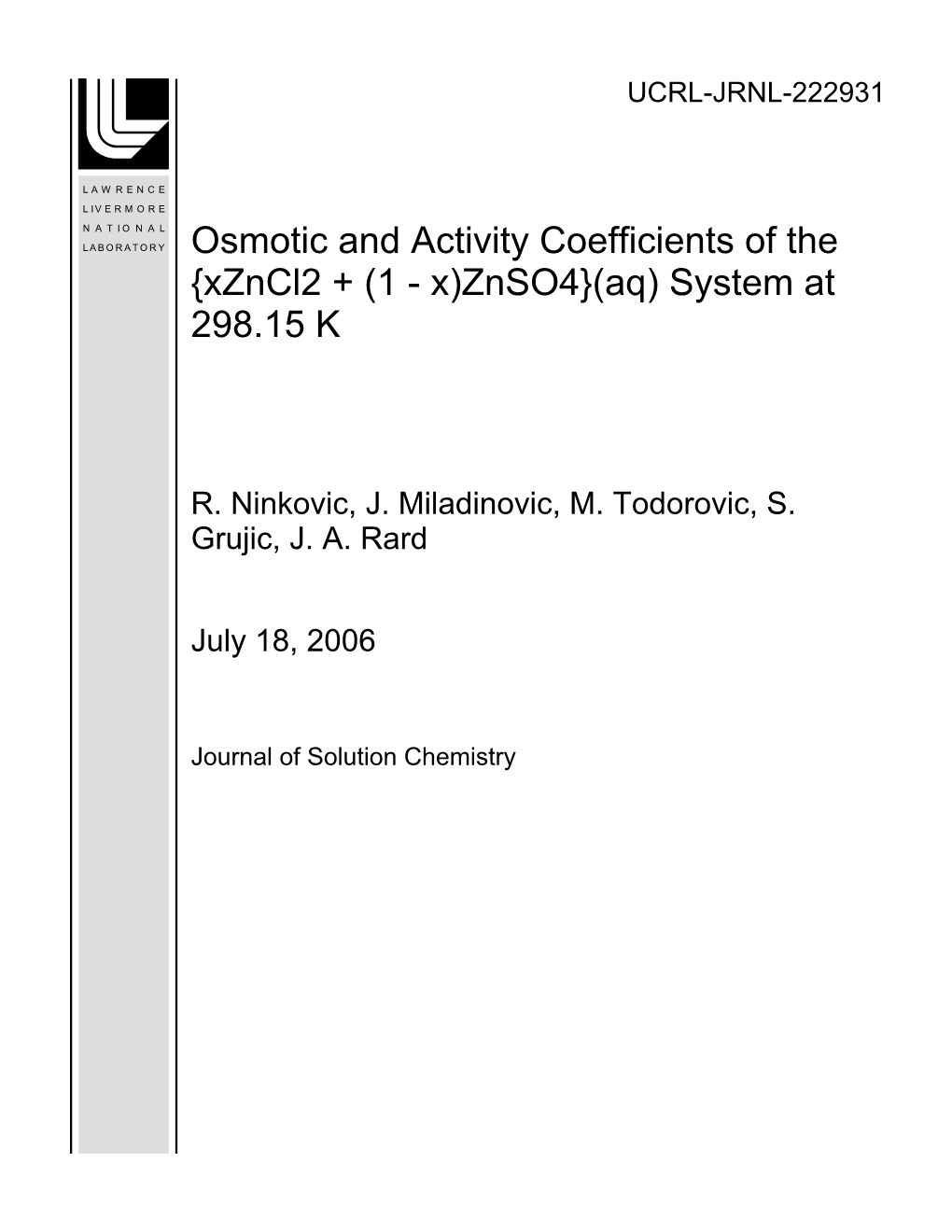
Load more
Recommended publications
-
Prediction of Osmotic Coefficients by Pair Correlation Function Method" (1991)
New Jersey Institute of Technology Digital Commons @ NJIT Dissertations Electronic Theses and Dissertations Spring 1-31-1991 Thermodynamics of strong electrolyte solutions : prediction of osmotic coefficientsy b pair correlation function method One Kwon Rim New Jersey Institute of Technology Follow this and additional works at: https://digitalcommons.njit.edu/dissertations Part of the Chemical Engineering Commons Recommended Citation Rim, One Kwon, "Thermodynamics of strong electrolyte solutions : prediction of osmotic coefficients by pair correlation function method" (1991). Dissertations. 1145. https://digitalcommons.njit.edu/dissertations/1145 This Dissertation is brought to you for free and open access by the Electronic Theses and Dissertations at Digital Commons @ NJIT. It has been accepted for inclusion in Dissertations by an authorized administrator of Digital Commons @ NJIT. For more information, please contact [email protected]. Copyright Warning & Restrictions The copyright law of the United States (Title 17, United States Code) governs the making of photocopies or other reproductions of copyrighted material. Under certain conditions specified in the law, libraries and archives are authorized to furnish a photocopy or other reproduction. One of these specified conditions is that the photocopy or reproduction is not to be “used for any purpose other than private study, scholarship, or research.” If a, user makes a request for, or later uses, a photocopy or reproduction for purposes in excess of “fair use” that user may be -
The Acidity of Atmospheric Particles and Clouds Havala O
The Acidity of Atmospheric Particles and Clouds Havala O. T. Pye1, Athanasios Nenes2,3, Becky Alexander4, Andrew P. Ault5, Mary C. Barth6, Simon L. Clegg7, Jeffrey L. Collett, Jr.8, Kathleen M. Fahey1, Christopher J. Hennigan9, Hartmut Herrmann10, Maria Kanakidou11, James T. Kelly12, I-Ting Ku8, V. Faye McNeill13, Nicole Riemer14, Thomas 5 Schaefer10, Guoliang Shi15, Andreas Tilgner10, John T. Walker1, Tao Wang16, Rodney Weber17, Jia Xing18, Rahul A. Zaveri19, Andreas Zuend20 1Office of Research and Development, U.S. Environmental Protection Agency, Research Triangle Park, NC, 27711, USA 2School of Architecture, Civil and Environmental Engineering, Ecole Polytechnique Fédérale de Lausanne, Lausanne, CH- 1015, Switzerland 10 3Institute for Chemical Engineering Sciences, Foundation for Research and Technology Hellas, Patras, GR-26504, Greece 4Department of Atmospheric Science, University of Washington, Seattle, WA, 98195, USA 5Department of Chemistry, University of Michigan, Ann Arbor, MI, 48109-1055, USA 6National Center for Atmospheric Research, Boulder, CO, 80307, USA 7School of Environmental Sciences, University of East Anglia, Norwich NR4 7TJ, UK 15 8Department of Atmospheric Science, Colorado State University, Fort Collins, CO, 80523, USA 9Department of Chemical, Biochemical, and Environmental Engineering, University of Maryland Baltimore County, Baltimore, MD, 21250, USA 10Leibniz Institute for Tropospheric Research (TROPOS), Atmospheric Chemistry Department (ACD), Leipzig, 04318, Germany 20 11Department of Chemistry, University -
Thermodynamic Properties of 1:1 Salt Aqueous Solutions with the Electrolattice M
D o s s i e r This paper is a part of the hereunder thematic dossier published in OGST Journal, Vol. 68, No. 2, pp. 187-396 and available online here Cet article fait partie du dossier thématique ci-dessous publié dans la revue OGST, Vol. 68, n°2, pp. 187-396 et téléchargeable ici © Photos: IFPEN, Fotolia, X. DOI: 10.2516/ogst/2012094 DOSSIER Edited by/Sous la direction de : Jean-Charles de Hemptinne InMoTher 2012: Industrial Use of Molecular Thermodynamics InMoTher 2012 : Application industrielle de la thermodynamique moléculaire Oil & Gas Science and Technology – Rev. IFP Energies nouvelles, Vol. 68 (2013), No. 2, pp. 187-396 Copyright © 2013, IFP Energies nouvelles 187 > Editorial électrostatique ab initio X. Rozanska, P. Ungerer, B. Leblanc and M. Yiannourakou 217 > Improving the Modeling of Hydrogen Solubility in Heavy Oil Cuts Using an Augmented Grayson Streed (AGS) Approach 309 > Improving Molecular Simulation Models of Adsorption in Porous Materials: Modélisation améliorée de la solubilité de l’hydrogène dans des Interdependence between Domains coupes lourdes par l’approche de Grayson Streed Augmenté (GSA) Amélioration des modèles d’adsorption dans les milieux poreux R. Torres, J.-C. de Hemptinne and I. Machin par simulation moléculaire : interdépendance entre les domaines J. Puibasset 235 > Improving Group Contribution Methods by Distance Weighting Amélioration de la méthode de contribution du groupe en pondérant 319 > Performance Analysis of Compositional and Modified Black-Oil Models la distance du groupe For a Gas Lift Process A. Zaitseva and V. Alopaeus Analyse des performances de modèles black-oil pour le procédé d’extraction par injection de gaz 249 > Numerical Investigation of an Absorption-Diffusion Cooling Machine Using M. -
Chaotic Temperatures Vs Coefficients of Thermodynamic Activity: the Advantage of the Method of Chemical Dynamics
CHAOTIC TEMPERATURES VS COEFFICIENTS OF THERMODYNAMIC ACTIVITY: THE ADVANTAGE OF THE METHOD OF CHEMICAL DYNAMICS B. Zilbergleyt Bank One, IT Department, Chicago E-mail: [email protected] ABSTRACT. The article compares traditional coefficients of thermodynamic activity as a parameter related to individual chemical species to newly introduced reduced chaotic temperatures as system characteristics, both regarding their usage in thermodynamic simulation of open chemical systems. Logical and mathematical backgrounds of both approaches are discussed. It is shown that usage of reduced chaotic temperatures and the Method of Chemical Dynamics to calculate chemical and phase composition in open chemical systems is much less costly, easier to perform and potentially leads to better precision. PROBABILISTIC MODEL OF COMPLEX CHEMICAL EQUILIBRIUM. It is well known how easily the Guldberg-Waage’s equation can be derived from the probabilistic considerations. Being based on the particle collisions, typical for the reactions in gases, it states that chance of the reaction to happen is proportional to joint probability P(A) of reactant particles to occur simultaneously at the same point of the reaction space. This probability merely equals to product of concentrations of the colliding particles. If multiplied by the rate constant it defines the reaction rate. When the rates of the forward and the reverse reactions are equal then the state of equilibrium exists. Less known is the fact that the above derivation is valid only in the case when no one of reactants can be consumed in any other collision with different outcome than A. Chemical system with only one type of collision represents isolated by the reactants (more traditionally referred to as closed) is a simple chemical system: one type of collision – one outcome. -
Chemical Equilibrium As Balance of the Thermodynamic Forces
Chemical Equilibrium as Balance of the Thermodynamic Forces B. Zilbergleyt, System Dynamics Research Foundation, Chicago, USA, E-mail: [email protected] ABSTRACT. The article sets forth comprehensive basics of thermodynamics of chemical equilibrium as balance of the thermodynamic forces. Based on the linear equations of irreversible thermodynamics, De Donder definition of the thermodynamic force, and Le Chatelier’s principle, our new theory of chemical equilibrium offers an explicit account for multiple chemical interactions within the system. Basic relations between energetic characteristics of chemical transformations and reaction extents are based on the idea of chemical equilibrium as balance between internal and external thermodynamic forces, which is presented in the form of a logistic equation. This equation contains only one new parameter, reflecting the external impact on the chemical system and the system’s resistance to potential changes. Solutions to the basic equation at isothermic-isobaric conditions define the domain of states of the chemical system, including four distinctive areas from true equilibrium to true chaos. The new theory is derived exclusively from the currently recognized ideas of chemical thermodynamics and covers both thermodynamics, equilibrium and non-equilibrium in a unique concept, bringing new opportunities for understanding and practical treatment of complex chemical systems. Among new features one should mention analysis of the system domain of states and the area limits, and a more accurate calculation of the equilibrium compositions. INTRODUCTION. Contemporary chemical thermodynamics is torn apart applying different concepts to traditional isolated systems with true thermodynamic equilibriumi and to open systems with self-organization, loosely described as “far-from-equilibrium” area. -
Thermodynamic Studies of the Fe-Pt System and “Feo”-Containing Slags for Application Towards Ladle Refining
Thermodynamic Studies of the Fe-Pt System and “FeO”-Containing Slags for Application Towards Ladle Refining Patrik Fredriksson Doctoral Dissertation Stockholm 2003 Royal Institute of Technology Department of Material Science and Engineering Division of Metallurgy Akademisk avhandling som med tillstånd av Kungliga Tekniska Högskolan i Stockholm, framlägges för offentlig granskning för avläggande av Teknologie doktorsexamen, fredagen den 7 November 2003, kl. 10.00 i Kollegiesalen, Administrationsbyggnaden, Kungliga Tekniska Högskolan, Valhallavägen 79 Stockholm ISRN KTH/MSE--03/36--SE+THMETU/AVH ISBN 91-7283-592-3 To Anna ii Abstract In the present work, the thermodynamic activites of iron oxide, denoted as “FeO” in the slag systems Al2O3-“FeO”, CaO-“FeO”, “FeO”-SiO2, Al2O3-“FeO”-SiO2, CaO- “FeO”-SiO2 and “FeO”-MgO-SiO2 were investigated by employing the gas equilibration technique at steelmaking temperatures. The strategy was to expose the molten slag mixtures kept in platinum crucibles for an oxygen potential, determined by a CO/CO2-ratio. A part of the iron reduced from the “FeO” in the slag phase was dissolved into the Pt crucible. In order to obtain the activites of “FeO”, chemical analysis of the quenched slag samples together with thermodynamic information of the binary metallic system Fe-Pt is required. Careful experimental work was carried out by employing a solid-state galvanic cell technique as well as calorimetric measurements in the temperature ranges of 1073-1273 K and 300-1988 K respectively. The outcome of these experiments was incorporated along with previous studies into a CALPHAD-type of thermodynamic assessment performed with the Thermo-Calc™ software. The proposed equilibrium diagram enabled extrapolation to higher temperatures. -
Measurement of Carbon Thermodynamic Activity In
J - carbon flow through sensor membrane 83 MEASUREMENT OF CARBON THERMODYNAMIC 2 ACTIVITY IN SODIUM g , cm" , min" ; t - time, s; GJT - sodium flow rate irr/hour, L/hour; F.A, KOZLOV, Yu.I. ZAGORULKO, Yu.P. KOVALEV, H_ - sensor signal, vol. % CH ; V.V. ALEKSEEV g 4 Institute of Physics and Power Engineering, Gr - decarburizing gas flow rate through sensor ; Obninsk, o Union of Soviet Socialist Republics T,t - t emperature. C; t - sensor t emperature; Gg - sodium flow rate through sensor. INTRODUCTION ABSTRACT Continuous detection of carbon thermodynaraic activity in The report presents the brief outline on system of carbon sodium coolant of energy installations with fast neutron reac- activity detecting system in sodium(SCD), operating on the tors presupposes conducting the following main functions; : carbon - permeable membrane, of the methods and the results of - Control of carburization sodium potential with reference to testing it under the experimental circulating loop conditions. construction materials; The results of carbon activity sensor calibration with the u,s,e - Control of sodium coolant accidental contamination by carbon- of equilibrium samples of XI8H9, Fe -8Ni, Pe ~12Mn materials bearing impurities, for example, as a result of oil leakage from are listed. The behawiour of carbon activity sensor signals in a centrifuginal pump cooling system. sogium under various transitional conditions and hydrodynamic Performing these functions under the monisothermal loop condi- perturbation in the circulating loop, containing carbon bearing tions has specific features which on the one hand depend on impurities in the sodium flow and their deposits on the surfa- construction and operating parameters of detectors and on the ces flushed by sodium, are described. -
THE CONCEPT of ELECTRON ACTIVITY and ITS RELATION to REDOX POTENTIALS in AQUEOUS GEOCHEMICAL SYSTEMS by Donald C
THE CONCEPT OF ELECTRON ACTIVITY AND ITS RELATION TO REDOX POTENTIALS IN AQUEOUS GEOCHEMICAL SYSTEMS by Donald C. Thorstenson U.S. GEOLOGICAL SURVEY Open-File Report 84 072 1984 UNITED STATES DEPARTMENT OF THE INTERIOR WILLIAM T. CLARK, Secretary GEOLOGICAL SURVEY Dallas L. Peck, Director For additional information Copies of this report may be write to: purchased from: Regional Research Hydrologist U.S. Geological Survey U.S. Geological Survey Western Distribution Branch Water Resources Division Open-File Services Section 432 National Center Box 25425, Federal Center Reston, Virginia 22092 Denver, Colorado 80225 ERRATA: (p. 1) 1. Equation (2), p. 3, should read: n 2.30RT 1 n 2.30RT , x = Ej? + _____ log__= E° + _____pH . (2) 2. Table 1, p. 6, entry 5, should read: In pure water, p e - 6.9; 3. Line 3, paragraph 3, p. 10 should read: As Fe^+ / aa \ is converted to Fe ( aa ) via equation (15) to 4. Equation (22), p. 13, should read: a n p - anya n+ e (aq) UA (aq) K = . (22) 5. Equation (38), p. 18, should read: F -log ae - = _______ Eh + . (38) ( acl) 2.303RT 2.303RT 6. Equation (52), p. 23, should read: ae- (aq) 7. Equation (53), p. 23, should read: pe s +6.9 and Eh s + 0.41 volt. (53) 8. Equation (54c), p. 23, should read: nu-'e ~ 10 -55.5 (aq) ERRATA: (p. 2) 9. The second line from the bottom, p. 28, should read: ....... (a state that has been maintained for * 105 years based on .... 10. The heading of Table 2, p. -
9.2 Raoult's Law and Henry's
Chapter 9 The Behavior of Solutions 1 9.2 Raoult’s Law and Henry’s Law (1) Initially evacuated vessel at T liq. A (2) spontaneously evaporate until P in the vessel ° reaches the saturated vapor pressure of liq. A, p A, at T (3) a dynamic equilibrium established between the rate of evaporation of liq. A & the rate of condensation of vapor A. 2 9.2 Raoult’s Law and Henry’s Law • re(A) α the # of A atoms in the vapor phase, ° thus, rc(A) = kp A , and at equilibrium (9.1) • Similarly, pure liquid B (9.2) re(A) rc(A) liq. A 3 9.2 Raoult’s Law and Henry’s Law (A) the effect of the small addition of liquid B to liquid A Assume (1) the composition of the surface of the liquid = that of the bulk liquid, XA, (2) the sizes of A & B atoms comparable (9.3) (9.4) 4 9.2 Raoult’s Law and Henry’s Law • From (9.1) and (9.3) gives (9.5) and from (9.2) and (9.4) gives (9.6) • Raoult’s law: the vapor pressure exerted by a component i in a solution = (the mole fraction of i in the solution) X (the saturated vapor pressure of pure liquid i ) at T of the solution. 5 9.2 Raoult’s Law and Henry’s Law • Requirements: the intrinsic rates of evaporation of A and B ≠ f(comp. of the solution) i.e., the magnitudes of the A-A, B-B, and A-B bond energies in the solution are identical 6 9.2 Raoult’s Law and Henry’s Law (B) If A-B >> A-A and B-B and a solution of A in B is sufficiently dilute: A atom at the surface surrounded only by B, A in a deeper potential E well than are the A atoms at the surface of pure liq… difficult to be lifted to vapor, evaporation rate is decreased to re(A) to re’(A) 7 9.2 Raoult’s Law and Henry’s Law (9.7) re(A) > re’(A) (9.8) ’ (9.9) • A similar consideration of dilute solutions of B in A gives ’ (9.10) • (9.9),(9.10) : Henry’ law 8 9.2 Raoult’s Law and Henry’s Law Beyond a critical value of XA, re’(A) becomes composition- dependent. -
Fugacity Nov 2 2011.Pdf
Fugacity - Wikipedia, the free encyclopedia 頁 1 / 5 Fugacity From Wikipedia, the free encyclopedia In chemical thermodynamics, the fugacity (f) of a real gas is an effective pressure which replaces the true mechanical pressure in accurate chemical equilibrium calculations. It is equal to the pressure of an ideal gas which has the same chemical potential as the real gas. For example, [1] nitrogen gas (N2) at 0°C and a pressure of 100 atm has a fugacity of 97.03 atm. This means that the chemical potential of real nitrogen at a pressure of 100 atm has the value which ideal nitrogen would have at a pressure of 97.03 atm. Fugacities are determined experimentally or estimated for various models such as a Van der Waals gas that are closer to reality than an ideal gas. The ideal gas pressure and fugacity are related through the dimensionless fugacity coefficient .[2] For nitrogen at 100 atm, the fugacity coefficient is 97.03 atm / 100 atm = 0.9703. For an ideal gas, fugacity and pressure are equal so is 1. The fugacity is closely related to the thermodynamic activity. For a gas, the activity is simply the fugacity divided by a reference pressure to give a dimensionless quantity. This reference pressure is called the standard state and normally chosen as 1 atmosphere or 1 bar, Again using nitrogen at 100 atm as an example, since the fugacity is 97.03 atm, the activity is just 97.03 with no units. Accurate calculations of chemical equilibrium for real gases should use the fugacity rather than the pressure. -
New Thermodynamic Activity-Based Approach Allows Predicting The
www.nature.com/scientificreports OPEN New thermodynamic activity‑based approach allows predicting the feasibility of glycolysis Thorsten Greinert1, Kristina Vogel2, Thomas Maskow2 & Christoph Held1* Thermodynamic feasibility analyses help evaluating the feasibility of metabolic pathways. This is an important information used to develop new biotechnological processes and to understand metabolic processes in cells. However, literature standard data are uncertain for most biochemical reactions yielding wrong statements concerning their feasibility. In this article we present activity‑based equilibrium constants for all the ten glycolytic reactions, accompanied by the standard reaction data (standard Gibbs energy of reaction and standard enthalpy of reaction). We further developed a thermodynamic activity‑based approach that allows to correctly determine the feasibility of glycolysis under diferent chosen conditions. The results show for the frst time that the feasibility of glycolysis can be explained by thermodynamics only if (1) correct standard data are used and if (2) the conditions in the cell at non‑equilibrium states are accounted for in the analyses. The results here will help to determine the feasibility of other metabolisms and to understand metabolic processes in cells in the future. Biotechnological routes ofer several advantages over classical chemical routes to produce chemicals, such as higher reaction selectivity and milder reaction conditions, yielding an overall more sustainable processes 1. Fur- thermore, such routes access a world full of new syntheses protocols using reactions that require the use of an enzyme or multiple enzymes in a complete metabolic pathway. However, fnding such new syntheses routes is generally expensive due to high experimental efort. So-called thermodynamic feasibility analyses (TFA) help to determine whether a metabolic pathway is generally feasible or not from thermodynamic point-of-view. -
CH. 9 Electrolyte Solutions
CH. 9 Electrolyte Solutions 9.1 Activity Coefficient of a Nonvolatile Solute in Solution and Osmotic Coefficient for the Solvent As shown in Ch. 6, the chemical potential is 0 where i is the chemical potential in the standard state and is a measure of concentration. For a nonvolatile solute, its pure liquid is often not a convenient standard state because a pure nonvolatile solute cannot exist as a liquid. For the dissolved solute, * i is the chemical potential of i in a hypothetical ideal solution at unit concentration (i = 1). In the ideal solution i = 1 for all compositions In real solution, i 1 as i 0 A common misconception: The standard state for the solute is the solute at infinite dilution. () At infinite dilution, the chemical potential of the solute approaches . Thus, the standard state should be at some non-zero concentration. A standard state need not be physically realizable, but it must be well- defined. For convenience, unit concentration i = 1 is used as the standard state. Three composition scales: Molarity (moles of solute per liter of solution, ci) The standard state of the solute is a hypothetical ideal 1-molar solution of i. (c) In real solution, i 1 as ci 0 Molality (moles of solute per kg of solvent, mi) // commonly used for electrolytes // density of solution not needed The standard state is hypothetical ideal 1-molal solution of i. (m) In real solution, i 1 as mi 0 Mole fraction xi Molality is an inconvenient scale for concentrated solution, and the mole fraction is a more convenient scale.