The Evolution of Mollusc Shells
Total Page:16
File Type:pdf, Size:1020Kb
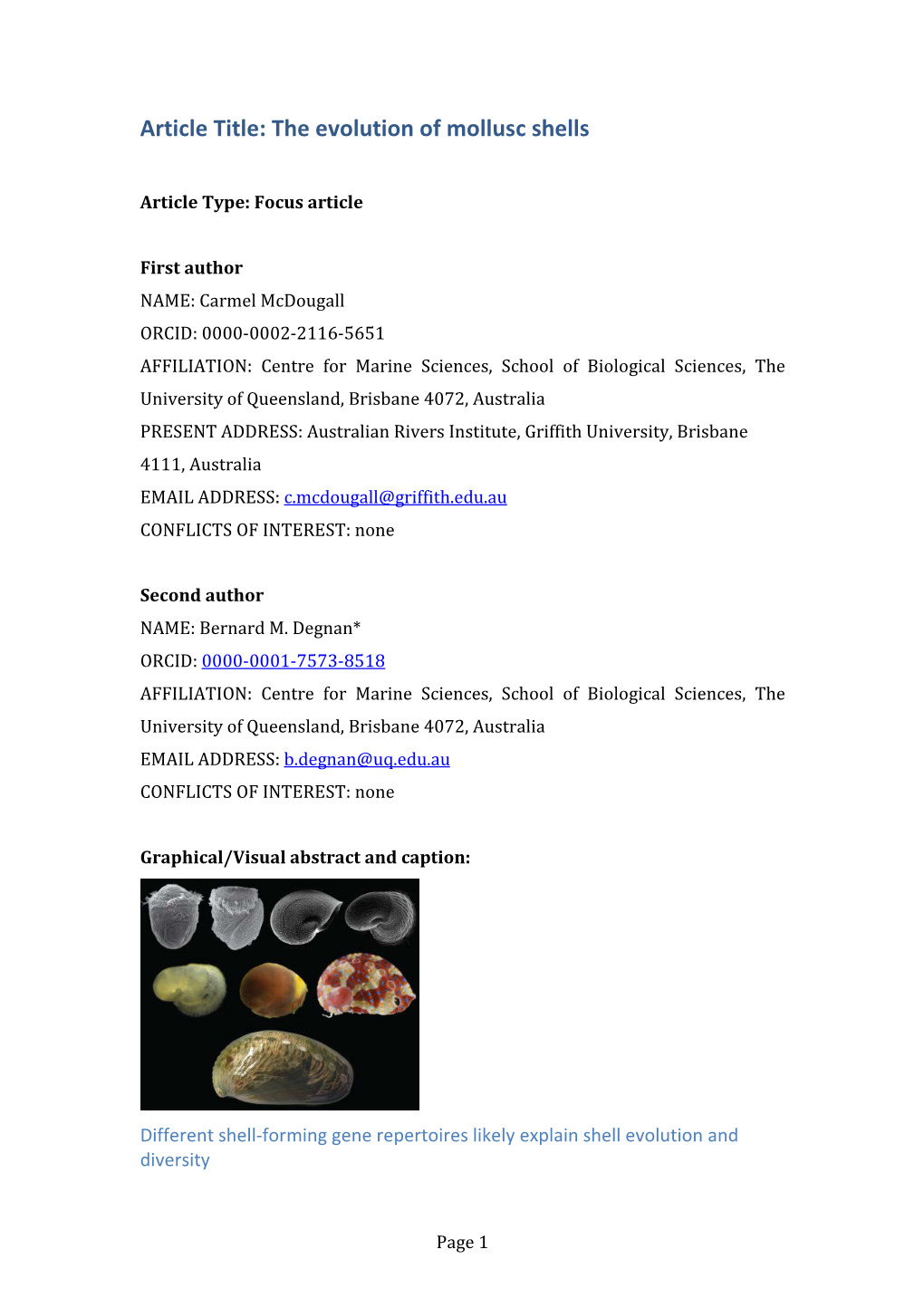
Load more
Recommended publications
-
Shell Morphology, Radula and Genital Structures of New Invasive Giant African Land
bioRxiv preprint doi: https://doi.org/10.1101/2019.12.16.877977; this version posted December 16, 2019. The copyright holder for this preprint (which was not certified by peer review) is the author/funder, who has granted bioRxiv a license to display the preprint in perpetuity. It is made available under aCC-BY 4.0 International license. 1 Shell Morphology, Radula and Genital Structures of New Invasive Giant African Land 2 Snail Species, Achatina fulica Bowdich, 1822,Achatina albopicta E.A. Smith (1878) and 3 Achatina reticulata Pfeiffer 1845 (Gastropoda:Achatinidae) in Southwest Nigeria 4 5 6 7 8 9 Alexander B. Odaibo1 and Suraj O. Olayinka2 10 11 1,2Department of Zoology, University of Ibadan, Ibadan, Nigeria 12 13 Corresponding author: Alexander B. Odaibo 14 E.mail :[email protected] (AB) 15 16 17 18 1 bioRxiv preprint doi: https://doi.org/10.1101/2019.12.16.877977; this version posted December 16, 2019. The copyright holder for this preprint (which was not certified by peer review) is the author/funder, who has granted bioRxiv a license to display the preprint in perpetuity. It is made available under aCC-BY 4.0 International license. 19 Abstract 20 The aim of this study was to determine the differences in the shell, radula and genital 21 structures of 3 new invasive species, Achatina fulica Bowdich, 1822,Achatina albopicta E.A. 22 Smith (1878) and Achatina reticulata Pfeiffer, 1845 collected from southwestern Nigeria and to 23 determine features that would be of importance in the identification of these invasive species in 24 Nigeria. -
Impacts of Ocean Acidification on Marine Shelled Molluscs
Mar Biol DOI 10.1007/s00227-013-2219-3 ORIGINAL PAPER Impacts of ocean acidification on marine shelled molluscs Fre´de´ric Gazeau • Laura M. Parker • Steeve Comeau • Jean-Pierre Gattuso • Wayne A. O’Connor • Sophie Martin • Hans-Otto Po¨rtner • Pauline M. Ross Received: 18 January 2013 / Accepted: 15 March 2013 Ó Springer-Verlag Berlin Heidelberg 2013 Abstract Over the next century, elevated quantities of ecosystem services including habitat structure for benthic atmospheric CO2 are expected to penetrate into the oceans, organisms, water purification and a food source for other causing a reduction in pH (-0.3/-0.4 pH unit in the organisms. The effects of ocean acidification on the growth surface ocean) and in the concentration of carbonate ions and shell production by juvenile and adult shelled molluscs (so-called ocean acidification). Of growing concern are the are variable among species and even within the same impacts that this will have on marine and estuarine species, precluding the drawing of a general picture. This organisms and ecosystems. Marine shelled molluscs, which is, however, not the case for pteropods, with all species colonized a large latitudinal gradient and can be found tested so far, being negatively impacted by ocean acidifi- from intertidal to deep-sea habitats, are economically cation. The blood of shelled molluscs may exhibit lower and ecologically important species providing essential pH with consequences for several physiological processes (e.g. respiration, excretion, etc.) and, in some cases, increased mortality in the long term. While fertilization Communicated by S. Dupont. may remain unaffected by elevated pCO2, embryonic and Fre´de´ric Gazeau and Laura M. -
Nautiloid Shell Morphology
MEMOIR 13 Nautiloid Shell Morphology By ROUSSEAU H. FLOWER STATEBUREAUOFMINESANDMINERALRESOURCES NEWMEXICOINSTITUTEOFMININGANDTECHNOLOGY CAMPUSSTATION SOCORRO, NEWMEXICO MEMOIR 13 Nautiloid Shell Morphology By ROUSSEAU H. FLOIVER 1964 STATEBUREAUOFMINESANDMINERALRESOURCES NEWMEXICOINSTITUTEOFMININGANDTECHNOLOGY CAMPUSSTATION SOCORRO, NEWMEXICO NEW MEXICO INSTITUTE OF MINING & TECHNOLOGY E. J. Workman, President STATE BUREAU OF MINES AND MINERAL RESOURCES Alvin J. Thompson, Director THE REGENTS MEMBERS EXOFFICIO THEHONORABLEJACKM.CAMPBELL ................................ Governor of New Mexico LEONARDDELAY() ................................................... Superintendent of Public Instruction APPOINTEDMEMBERS WILLIAM G. ABBOTT ................................ ................................ ............................... Hobbs EUGENE L. COULSON, M.D ................................................................. Socorro THOMASM.CRAMER ................................ ................................ ................... Carlsbad EVA M. LARRAZOLO (Mrs. Paul F.) ................................................. Albuquerque RICHARDM.ZIMMERLY ................................ ................................ ....... Socorro Published February 1 o, 1964 For Sale by the New Mexico Bureau of Mines & Mineral Resources Campus Station, Socorro, N. Mex.—Price $2.50 Contents Page ABSTRACT ....................................................................................................................................................... 1 INTRODUCTION -
Haliotis Asinina) in Coastal Waters of Thailand Determined Using Microsatellite Markers
Mar. Biotechnol. 6, 604–611, 2004 DOI: 10.1007/s10126-004-2300-5 Ó 2005 Springer Science+Business Media, Inc. Population Structure of Tropical Abalone (Haliotis asinina) in Coastal Waters of Thailand Determined Using Microsatellite Markers S. Tang,1 A. Tassanakajon,1 S. Klinbunga,2 P. Jarayabhand,3,4 and P. Menasveta2,4 1Department of Biochemistry, Faculty of Science, Chulalongkorn University, Bangkok 10330, Thailand 2Marine Biotechnology Research Unit, National Center for Genetic Engineering and Biotechnology (BIOTEC), National Science and Technology Development Agency, Pathumthani 12120, Thailand 3Aquatic Resources Research Institute, Chulalongkorn University, Bangkok 10330, Thailand 4Department of Marine Science, Faculty of Science, Chulalongkorn University, Bangkok 10330, Thailand Abstract: Three partial genomic libraries were constructed from genomic DNA of the tropical abalone (Haliotis asinina) that was digested with AluI, vortexed/sonicated, and digested with mixed enzyme (AluI, HincII, and RsaI). The libraries yielded 0.02%, 0.42%, and 1.46% positive microsatellite-containing clones, respectively. Eleven clones each of perfect, imperfect, and compound microsatellites were isolated. Ten primer pairs (CU- Has1–CUHas10) were analyzed to evaluate their polymorphic level. The numbers of alleles per locus, observed heterozygosity (H0), and expected heterozygosity (He) ranged from 3 to 26 alleles, and varied between 0.27 and 0.85 and between 0.24 and 0.93, respectively. Three microsatellite loci (CUHas2, CUHas3, and CUHas8) were further used for examination of genetic diversity and differentiation of natural H. asinina in coastal waters of Thailand. Genetic variabilities in terms of the effective number of alleles (ne), H0, and He were higher in 2 samples from the Gulf of Thailand (ne = 9.37, 7.66; H0 = 0.62, 0.78; and He = 0.87, 0.86) than those of one sample (ne = 6.04; H0 = 0.58; and He = 0.62) derived from the Andaman Sea. -
Download Book (PDF)
M o Manual on IDENTIFICATION OF SCHEDULE MOLLUSCS From India RAMAKRISHN~~ AND A. DEY Zoological Survey of India, M-Block, New Alipore, Kolkota 700 053 Edited by the Director, Zoological Survey of India, Kolkata ZOOLOGICAL SURVEY OF INDIA KOLKATA CITATION Ramakrishna and Dey, A. 2003. Manual on the Identification of Schedule Molluscs from India: 1-40. (Published : Director, Zool. Surv. India, Kolkata) Published: February, 2003 ISBN: 81-85874-97-2 © Government of India, 2003 ALL RIGHTS RESERVED • No part of this publication may be reproduced, stored in a retrieval system or transmitted, in any from or by any means, electronic, mechanical, photocopying, recording or otherwise without the prior permission of the publisher. • -This book is sold subject to the condition that it shall not, by way of trade, be lent, resold hired out or otherwise disposed of without the publisher's consent, in any form of binding or cover other than that in which it is published. • The correct price of this publication is the price printed on this page. Any revised price indicated by a rubber stamp or by a sticker or by any other means is incorrect and should be unacceptable. PRICE India : Rs. 250.00 Foreign : $ (U.S.) 15, £ 10 Published at the Publication Division by the Director, Zoological Survey of India, 234/4, AJ.C. Bose Road, 2nd MSO Building (13th Floor), Nizam Palace, Kolkata -700020 and printed at Shiva Offset, Dehra Dun. Manual on IDENTIFICATION OF SCHEDULE MOLLUSCS From India 2003 1-40 CONTENTS INTRODUcrION .............................................................................................................................. 1 DEFINITION ............................................................................................................................ 2 DIVERSITY ................................................................................................................................ 2 HA.B I,.-s .. .. .. 3 VAWE ............................................................................................................................................ -
Morphology and Systematic Position of Tryhlidium Canadense Whiteaves
Morphology and systematic position of Tryblidium canadense Whiteaves, 1884 (Mollusca) from the Silurian of North America JOHNS. PEEL Peel, J. S.: Morphology and systematic position of Tryblidium Canadense Whiteaves, 1884 (Mollusca) from the Silurian of North America. Bull. geol. Soc. Denmark, vol. 38, pp. 43-51. Copenhagen, April 25th, 1990. https://doi.org/10.37570/bgsd-1990-38-04 The nomenclative history of Tryblidium canadense Whiteaves, 1884, a large, oval, univalved mollusc originally described from the Silurian Guelph Formation of Ontario, is reviewed. Following comparison to Archinace/la Ulrich & Scofield, 1897, in which genus it has generally been placed for almost a century, Whiteaves' species is redescribed and assigned to a new gastropod genus, Guelphinace/la. John S. Peel, Geological Survey of Greenland, Oster Voldgade JO, 1350 Copenhagen K, Denmark. February 10th, 1989. Whiteaves (1884) described a single internal the sub-apical wall. He commented that the mould of a large (45 mm), oval, univalved mol structure seemed to be a single continuous mus lusc from the Guelph Formation (Silurian) of cular impression and not two separate depres Hespeler, Ontario, Canada as Tryblidium Cana sions, as suggested by Lindstrom (1884), al dense (Fig. 1). Uncertainty surrounding its sys though he did not refer directly to the latter's tematic position developed immediately when description. He made no reference to the thin Lindstrom (1884) questioned the assignment to dorsal band which Lindstrom (1884) had consid the genus Tryblidium -
Shell Microstructures in Early Cambrian Molluscs
Shell microstructures in Early Cambrian molluscs ARTEM KOUCHINSKY Kouchinsky, A. 2000. Shell microstructures in Early Cambrian molluscs. - Acta Palaeontologica Polonica 45,2, 119-150. The affinities of a considerable part of the earliest skeletal fossils are problematical, but investigation of their microstructures may be useful for understanding biomineralization mechanisms in early metazoans and helpful for their taxonomy. The skeletons of Early Cambrian mollusc-like organisms increased by marginal secretion of new growth lamel- lae or sclerites, the recognized basal elements of which were fibers of apparently aragon- ite. The juvenile part of some composite shells consisted of needle-like sclerites; the adult part was built of hollow leaf-like sclerites. A layer of mineralized prism-like units (low aragonitic prisms or flattened spherulites) surrounded by an organic matrix possibly existed in most of the shells with continuous walls. The distribution of initial points of the prism-like units on a periostracurn-like sheet and their growth rate were mostly regular. The units may be replicated on the surface of internal molds as shallow concave poly- gons, which may contain a more or less well-expressed tubercle in their center. Tubercles are often not enclosed in concave polygons and may co-occur with other types of tex- tures. Convex polygons seem to have resulted from decalcification of prism-like units. They do not co-occur with tubercles. The latter are interpreted as casts of pore channels in the wall possibly playing a role in biomineralization or pits serving as attachment sites of groups of mantle cells. Casts of fibers and/or lamellar units may overlap a polygonal tex- ture or occur without it. -
Predatory Poiretia (Stylommatophora, Oleacinidae) Snails: Histology and Observations
Vita Malacologica 13: 35-48 20 December 2015 Predatory Poiretia (Stylommatophora, Oleacinidae) snails: histology and observations Renate A. HELWERDA Naturalis Biodiversity Center, Darwinweg 2, 2333 CR Leiden, The Netherlands email: [email protected] Key words: Predation, predatory snails, drilling holes, radula, pedal gland, sole gland, acidic mucus ABSTRACT The Mediterranean species occur in rather dry, often rocky habitats, which are openly to sparsely vegetated. The predatory behaviour of Poiretia snails is studied. One However, they also occur in anthropogenically affected areas aspect of this behaviour is the ability to make holes in the such as gardens and parks (Kittel, 1997). The snails are main - shells of prey snails. The radula and the histology of the ly active at night and are hidden away under rocks and leaf mucous glands support the assumption that Poiretia secretes litter during the day, although they can also be found crawling acidic mucus to produce these holes. Observation of a around during daytime if the weather is rainy or cloudy and Poiretia compressa (Mousson, 1859) specimen yielded the moist (Wagner, 1952; Maassen, 1977; Kittel, 1997). During insight that its activities relied on the availability of moisture the hot summer months, Poiretia snails aestivate by burying and not on light conditions. It preyed on a wide range of snail themselves in soil or under rocks and sealing their apertures species, but only produced holes in shells when the aperture with an epiphragm (Kittel, 1997). was blocked. It usually stabbed its prey with a quick motion Poiretia snails prey on a wide variety of pulmonate snails. -
Um Éon De História Dos Bivalvia: Ideias Sobre a Sua Origem, Filogenia E Importância Paleontológica E Educativa
Um Éon de história dos Bivalvia: ideias sobre a sua origem, filogenia e importância paleontológica e educativa Ricardo J. Pimentel1, Pedro M. Callapez2 & Paulo Legoinha3 1 Agrupamento de Escolas de Guia, P-3105 075 Guia, Pombal, Portugal. E-mail: [email protected] 2 Universidade de Coimbra, CITEUC - Centro de Investigação da Terra e do Espaço da Universidade de Coimbra, Faculdade de Ciências e Tecnologia, Departamento de Ciências da Terra, Polo II, Rua Sílvio Lima, P-3030 790 Coimbra, Portugal. E-mail: [email protected] 3 Universidade NOVA de Lisboa, GEOBIOTEC - GeoBiociências, Geotecnologias e Geoengenharias, Faculdade de Ciências e Tecnologia, Departamento de Ciências da Terra, Quinta da Torre, P-2829 516 Caparica, Portugal. E-mail: [email protected] Resumo: A Classe Bivalvia, cuja monofilia se encontra bem suportada por estudos recentes, destaca-se como o segundo taxon, em termos de biodiversidade, do Filo Mollusca. O registo da sua história evolutiva remonta ao Fortuniano (Câmbrico). Diversos dados paleontológicos fundamentados no registo fóssil sugerem que os bivalves atuais são, sobretudo, herdeiros de carateres morfológicos e requisitos ecológicos transmitidos por linhagens da transição devónico-carbonífera. Estes invertebrados habitam a maioria dos ambientes aquáticos atuais e constituem um dos principais grupos de animais invertebrados da biosfera atual, são herdeiros de uma longa história evolutiva e ecológica que é intrínseca à própria história biótica dos oceanos durante o Fanerozoico. São um exemplo de sucesso entre as comunidades bióticas e a sua abundância, diversidade e acessibilidade propiciam a sua utilização como recursos educativos em Ciências Naturais. Palavras-chave: Bivalvia, Educação científica, Filogenia, Mollusca, Registo fóssil. -
Fauna of New Zealand Ko Te Aitanga Pepeke O Aotearoa
aua o ew eaa Ko te Aiaga eeke o Aoeaoa IEEAE SYSEMAICS AISOY GOU EESEAIES O ACAE ESEAC ema acae eseac ico Agicuue & Sciece Cee P O o 9 ico ew eaa K Cosy a M-C aiièe acae eseac Mou Ae eseac Cee iae ag 917 Aucka ew eaa EESEAIE O UIESIIES M Emeso eame o Eomoogy & Aima Ecoogy PO o ico Uiesiy ew eaa EESEAIE O MUSEUMS M ama aua Eiome eame Museum o ew eaa e aa ogaewa O o 7 Weigo ew eaa EESEAIE O OESEAS ISIUIOS awece CSIO iisio o Eomoogy GO o 17 Caea Ciy AC 1 Ausaia SEIES EIO AUA O EW EAA M C ua (ecease ue 199 acae eseac Mou Ae eseac Cee iae ag 917 Aucka ew eaa Fauna of New Zealand Ko te Aitanga Pepeke o Aotearoa Number / Nama 38 Naturalised terrestrial Stylommatophora (Mousca Gasooa Gay M ake acae eseac iae ag 317 amio ew eaa 4 Maaaki Whenua Ρ Ε S S ico Caeuy ew eaa 1999 Coyig © acae eseac ew eaa 1999 o a o is wok coee y coyig may e eouce o coie i ay om o y ay meas (gaic eecoic o mecaica icuig oocoyig ecoig aig iomaio eiea sysems o oewise wiou e wie emissio o e uise Caaoguig i uicaio AKE G Μ (Gay Micae 195— auase eesia Syommaooa (Mousca Gasooa / G Μ ake — ico Caeuy Maaaki Weua ess 1999 (aua o ew eaa ISS 111-533 ; o 3 IS -7-93-5 I ie 11 Seies UC 593(931 eae o uIicaio y e seies eio (a comee y eo Cosy usig comue-ase e ocessig ayou scaig a iig a acae eseac M Ae eseac Cee iae ag 917 Aucka ew eaa Māoi summay e y aco uaau Cosuas Weigo uise y Maaaki Weua ess acae eseac O o ico Caeuy Wesie //wwwmwessco/ ie y G i Weigo o coe eoceas eicuaum (ue a eigo oaa (owe (IIusao G M ake oucio o e coou Iaes was ue y e ew eaIa oey oa ue oeies eseac -
Evolution of Large Body Size in Abalones (Haliotis): Patterns and Implications
Paleobiology, 31(4), 2005, pp. 591±606 Evolution of large body size in abalones (Haliotis): patterns and implications James A. Estes, David R. Lindberg, and Charlie Wray Abstract.ÐKelps and other ¯eshy macroalgaeÐdominant reef-inhabiting organisms in cool seasÐ may have radiated extensively following late Cenozoic polar cooling, thus triggering a chain of evolutionary change in the trophic ecology of nearshore temperate ecosystems. We explore this hypothesis through an analysis of body size in the abalones (Gastropoda; Haliotidae), a widely distributed group in modern oceans that displays a broad range of body sizes and contains fossil representatives from the late Cretaceous (60±75 Ma). Geographic analysis of maximum shell length in living abalones showed that small-bodied species, while most common in the Tropics, have a cosmopolitan distribution, whereas large-bodied species occur exclusively in cold-water ecosys- tems dominated by kelps and other macroalgae. The phylogeography of body size evolution in extant abalones was assessed by constructing a molecular phylogeny in a mix of large and small species obtained from different regions of the world. This analysis demonstrates that small body size is the plesiomorphic state and largeness has likely arisen at least twice. Finally, we compiled data on shell length from the fossil record to determine how (slowly or suddenly) and when large body size arose in the abalones. These data indicate that large body size appears suddenly at the Miocene/Pliocene boundary. Our ®ndings support the view that ¯eshy-algal dominated ecosys- tems radiated rapidly in the coastal oceans with the onset of the most recent glacial age. -
The Slit Bearing Nacreous Archaeogastropoda of the Triassic Tropical Reefs in the St
Berliner paläobiologische Abhandlungen 10 5-47 Berlin 2009-11-11 The slit bearing nacreous Archaeogastropoda of the Triassic tropical reefs in the St. Cassian Formation with evaluation of the taxonomic value of the selenizone Klaus Bandel Abstract: Many Archaeogastropoda with nacreous shell from St. Cassian Formation have a slit in the outer lip that gives rise to a selenizone. The primary objective of this study is to analyze family level characters, provide a revision of some generic classifications and compare with species living today. Members of twelve families are recognized with the Lancedellidae n. fam., Rhaphistomellidae n. fam., Pseudowortheniellidae n. fam., Pseudoschizogoniidae n. fam., Wortheniellidae n. fam. newly defined. While the organization of the aperture and the shell structure is similar to that of the living Pleurotomariidae, morphology of the early ontogenetic shell and size and shape of the adult shell distinguish the Late Triassic slit bearing Archae- gastropoda from these. In the reef environment of the tropical Tethys Ocean such Archaeogastropoda were much more diverse than modern representatives of that group from the tropical Indo-Pacific Ocean. Here Haliotis, Seguenzia and Fossarina represent living nacreous gastropods with slit and are compared to the fossil species. All three have distinct shape and arrangement of the teeth in their radula that is not related to that of the Pleurotomariidae and also differs among each other. The family Fossarinidae n. fam. and the new genera Pseudowortheniella and Rinaldoella are defined, and a new species Campbellospira missouriensis is described. Zusammenfassung: In der St. Cassian-Formation kommen zahlreiche Arten der Archaeogastropoda vor, die eine perlmutterige Schale mit Schlitz in der Außenlippe haben, welcher zu einem Schlitzband führt.