Reef Fish Larvae: Insights from Morphology
Total Page:16
File Type:pdf, Size:1020Kb
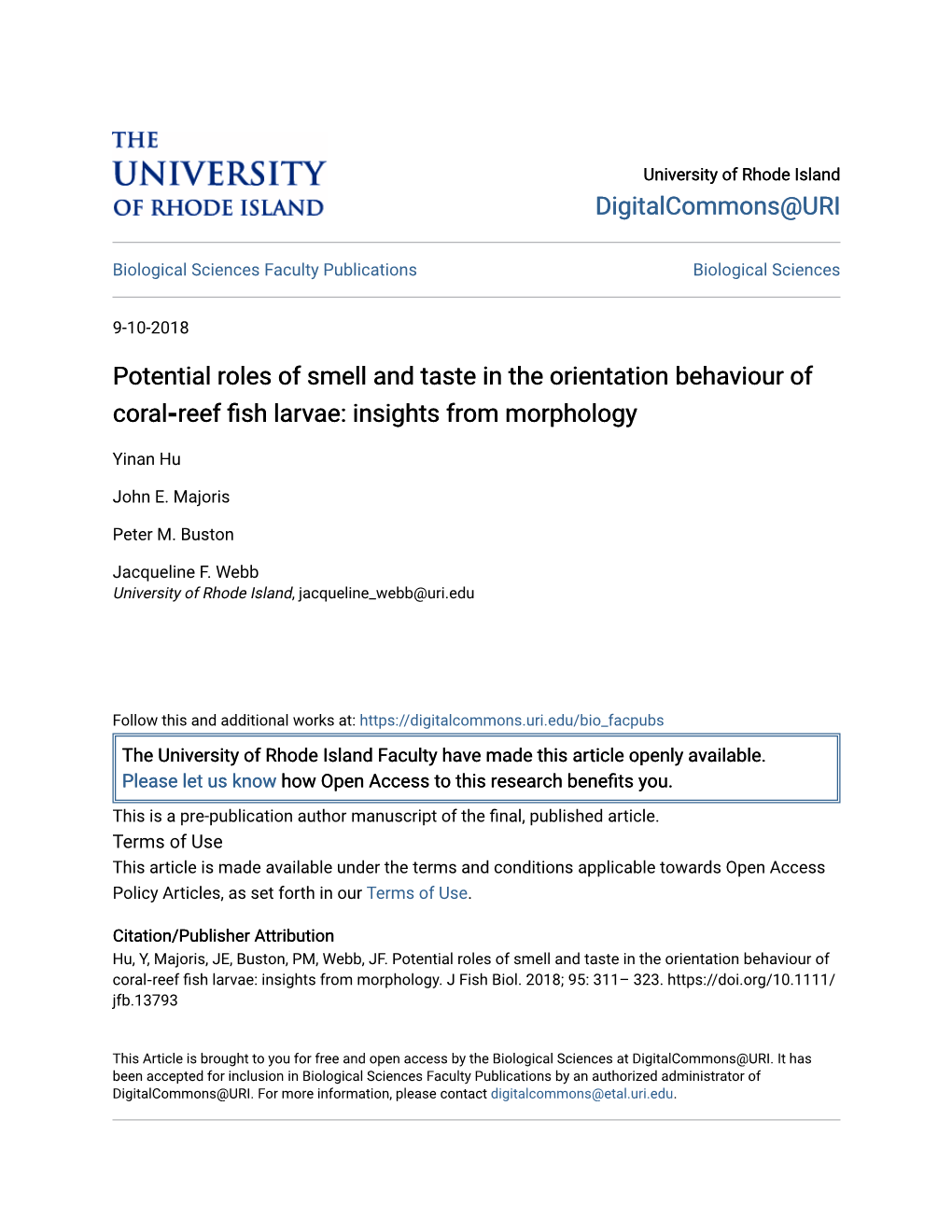
Load more
Recommended publications
-
The Reef Corridor of the Southwest Gulf of Mexico: Challenges for Its Management and Conservation
Ocean & Coastal Management 86 (2013) 22e32 Contents lists available at ScienceDirect Ocean & Coastal Management journal homepage: www.elsevier.com/locate/ocecoaman The Reef Corridor of the Southwest Gulf of Mexico: Challenges for its management and conservation Leonardo Ortiz-Lozano a,*, Horacio Pérez-España b,c, Alejandro Granados-Barba a, Carlos González-Gándara d, Ana Gutiérrez-Velázquez e, Javier Martos d a Análisis y Síntesis de Zonas Costeras, Instituto de Ciencias Marinas y Pesquerías, Universidad Veracruzana, Av. Hidalgo #617, Col. Río Jamapa 94290, Boca del Río, Veracruz, Mexico b Arrecifes Coralinos, Instituto de Ciencias Marinas y Pesquerías, Universidad Veracruzana, Av. Hidalgo #617, Col. Río Jamapa 94290, Boca del Río, Veracruz, Mexico c Centro de Investigación de Ciencias Ambientales, Universidad Autónoma del Carmen, Av. Laguna de Términos s/n, Col. Renovación 2da sección, CP 24155, Ciudad del Carmen, Campeche, Mexico d Laboratorio de Arrecifes Coralinos, Facultad de Ciencias Biológicas y Agropecuarias, Zona Poza Rica Tuxpan, Universidad Veracruzana, Carr. Tuxpan.- Tampico km 7.5, Col. Universitaria, CP 92860, Tuxpan, Veracruz, Mexico e Posgrado. Instituto de Ecología, A.C., Departamento de Ecología y Comportamiento Animal, Apartado Postal 63, Xalapa 91000, Veracruz, Mexico article info abstract Article history: Flow of species and spatial continuity of biological processes between geographically separated areas Available online may be achieved using management tools known as Ecological Corridors (EC). In this paper we propose an EC composed of three highly threatened coral reef systems in the Southwest Gulf of Mexico: Sistema Arrecifal Lobos Tuxpan, Sistema Arrecifal Veracruzano and Arrecifes de los Tuxtlas. The proposed EC is supported by the concept of Marine Protected Areas Networks, which highlights the biogeographical and habitat heterogeneity representations as the main criteria to the establishment of this kind of networks. -
Understanding Transformative Forces of Aquaculture in the Marine Aquarium Trade
The University of Maine DigitalCommons@UMaine Electronic Theses and Dissertations Fogler Library Summer 8-22-2020 Senders, Receivers, and Spillover Dynamics: Understanding Transformative Forces of Aquaculture in the Marine Aquarium Trade Bryce Risley University of Maine, [email protected] Follow this and additional works at: https://digitalcommons.library.umaine.edu/etd Part of the Marine Biology Commons Recommended Citation Risley, Bryce, "Senders, Receivers, and Spillover Dynamics: Understanding Transformative Forces of Aquaculture in the Marine Aquarium Trade" (2020). Electronic Theses and Dissertations. 3314. https://digitalcommons.library.umaine.edu/etd/3314 This Open-Access Thesis is brought to you for free and open access by DigitalCommons@UMaine. It has been accepted for inclusion in Electronic Theses and Dissertations by an authorized administrator of DigitalCommons@UMaine. For more information, please contact [email protected]. SENDERS, RECEIVERS, AND SPILLOVER DYNAMICS: UNDERSTANDING TRANSFORMATIVE FORCES OF AQUACULTURE IN THE MARINE AQUARIUM TRADE By Bryce Risley B.S. University of New Mexico, 2014 A THESIS Submitted in Partial Fulfillment of the Requirements for the Degree of Master of Science (in Marine Policy and Marine Biology) The Graduate School The University of Maine May 2020 Advisory Committee: Joshua Stoll, Assistant Professor of Marine Policy, Co-advisor Nishad Jayasundara, Assistant Professor of Marine Biology, Co-advisor Aaron Strong, Assistant Professor of Environmental Studies (Hamilton College) Christine Beitl, Associate Professor of Anthropology Douglas Rasher, Senior Research Scientist of Marine Ecology (Bigelow Laboratory) Heather Hamlin, Associate Professor of Marine Biology No photograph in this thesis may be used in another work without written permission from the photographer. -
A Dissertation Entitled Evolution, Systematics
A Dissertation Entitled Evolution, systematics, and phylogeography of Ponto-Caspian gobies (Benthophilinae: Gobiidae: Teleostei) By Matthew E. Neilson Submitted as partial fulfillment of the requirements for The Doctor of Philosophy Degree in Biology (Ecology) ____________________________________ Adviser: Dr. Carol A. Stepien ____________________________________ Committee Member: Dr. Christine M. Mayer ____________________________________ Committee Member: Dr. Elliot J. Tramer ____________________________________ Committee Member: Dr. David J. Jude ____________________________________ Committee Member: Dr. Juan L. Bouzat ____________________________________ College of Graduate Studies The University of Toledo December 2009 Copyright © 2009 This document is copyrighted material. Under copyright law, no parts of this document may be reproduced without the expressed permission of the author. _______________________________________________________________________ An Abstract of Evolution, systematics, and phylogeography of Ponto-Caspian gobies (Benthophilinae: Gobiidae: Teleostei) Matthew E. Neilson Submitted as partial fulfillment of the requirements for The Doctor of Philosophy Degree in Biology (Ecology) The University of Toledo December 2009 The study of biodiversity, at multiple hierarchical levels, provides insight into the evolutionary history of taxa and provides a framework for understanding patterns in ecology. This is especially poignant in invasion biology, where the prevalence of invasiveness in certain taxonomic groups could -
First Chromosome Analysis of the Humpback Cardinalfish, Fibramia
© 2017 The Japan Mendel Society Cytologia 82(1) Special Issue: 9–15 First Chromosome Analysis of the Humpback Cardinalfish, Fibramia lateralis (Perciformes, Apogonidae) Wannapa Kasiroek1,2, Chantra Indananda3, Nattawut Luangoon2, Krit Pinthong4, Weerayuth Supiwong5 and Alongklod Tanomtong6* 1 Department of Aquatic Science, Faculty of Science, Burapha University, Muang, Chonburi 20131, Thailand 2 Institute of Marine Science, Burapha University, Muang, Chonburi 20131, Thailand 3 Department of Biology, Faculty of Science, Burapha University, Muang, Chonburi 20131, Thailand 4 Department of Fundamental Science, Faculty of Science and Technology, Surindra Rajabhat University, Muang, Surin 32000, Thailand 5 Faculty of Applied Science and Engineering, Khon Kaen University, Nong Khai Campus, Muang, Nong Khai 43000, Thailand 6 Toxic Substances in Livestock and Aquatic Animals Research Group, Department of Biology, Faculty of Science, Khon Kaen University, Muang, Khon Kaen 40002, Thailand Received July 27, 2015; accepted November 23, 2015 Summary The first chromosome analysis and nucleolar organizer region (NOR) pattern of the humpback car- dinalfish (Fibramia lateralis) were studied. Samples from 10 male and 10 female fish were collected from the Andaman Sea and Gulf of Thailand. Mitotic chromosome preparations were prepared directly from kidney tis- sues. Conventional and Ag-NOR staining techniques were applied to stain the chromosomes. The results showed that the diploid chromosome number of F. lateralis was 2n=46, and the fundamental numbers (NF) were 54 in both sexes. The karyotype consisted of 8 large acrocentric, 12 large telocentric, 24 medium telocentric and 2 small telocentric chromosomes. Moreover, the results indicated that the region adjacent to the telomere of the short arm of the second acrocentric chromosome pair showed clearly observable nucleolar organizer regions (NORs). -
A List of Common and Scientific Names of Fishes from the United States And
t a AMERICAN FISHERIES SOCIETY QL 614 .A43 V.2 .A 4-3 AMERICAN FISHERIES SOCIETY Special Publication No. 2 A List of Common and Scientific Names of Fishes -^ ru from the United States m CD and Canada (SECOND EDITION) A/^Ssrf>* '-^\ —---^ Report of the Committee on Names of Fishes, Presented at the Ei^ty-ninth Annual Meeting, Clearwater, Florida, September 16-18, 1959 Reeve M. Bailey, Chairman Ernest A. Lachner, C. C. Lindsey, C. Richard Robins Phil M. Roedel, W. B. Scott, Loren P. Woods Ann Arbor, Michigan • 1960 Copies of this publication may be purchased for $1.00 each (paper cover) or $2.00 (cloth cover). Orders, accompanied by remittance payable to the American Fisheries Society, should be addressed to E. A. Seaman, Secretary-Treasurer, American Fisheries Society, Box 483, McLean, Virginia. Copyright 1960 American Fisheries Society Printed by Waverly Press, Inc. Baltimore, Maryland lutroduction This second list of the names of fishes of The shore fishes from Greenland, eastern the United States and Canada is not sim- Canada and the United States, and the ply a reprinting with corrections, but con- northern Gulf of Mexico to the mouth of stitutes a major revision and enlargement. the Rio Grande are included, but those The earlier list, published in 1948 as Special from Iceland, Bermuda, the Bahamas, Cuba Publication No. 1 of the American Fisheries and the other West Indian islands, and Society, has been widely used and has Mexico are excluded unless they occur also contributed substantially toward its goal of in the region covered. In the Pacific, the achieving uniformity and avoiding confusion area treated includes that part of the conti- in nomenclature. -
Interacting Effects of Elevated Temperature and Ocean Acidification on the Aerobic Performance of Coral Reef Fishes
Vol. 388: 235–242, 2009 MARINE ECOLOGY PROGRESS SERIES Published August 19 doi: 10.3354/meps08137 Mar Ecol Prog Ser Interacting effects of elevated temperature and ocean acidification on the aerobic performance of coral reef fishes Philip L. Munday1, 2,*, Natalie E. Crawley1, 2, Göran E. Nilsson3 1ARC Centre of Excellence for Coral Reef Studies, James Cook University, Townsville 4811, Australia 2School of Marine and Tropical Biology, James Cook University, Townsville 4811, Australia 3Physiology Programme, Department of Molecular Biosciences, University of Oslo, PO Box 1041, 0316 Oslo, Norway ABSTRACT: Concerns about the impacts of ocean acidification on marine life have mostly focused on how reduced carbonate saturation affects calcifying organisms. Here, we show that levels of CO2-induced acidification that may be attained by 2100 could also have significant effects on marine organisms by reducing their aerobic capacity. The effects of temperature and acidification on oxygen consumption were tested in 2 species of coral reef fishes, Ostorhinchus doederleini and O. cyanosoma, from the Great Barrier Reef, Australia. The capacity for aerobic activity (aerobic scope) declined at temperatures above the summer average (29°C) and in CO2-acidified water (pH 7.8 and ~1000 ppm CO2) compared to control water (pH 8.15). Aerobic scope declined by 36 and 32% for O. doederleini and O. cyanosoma at temperatures between 29 to 32°C, whereas it declined by 33 and 47% for O. doederleini and O. cyanosoma in acidified water compared to control water. Thus, the declines in aerobic scope in acidified water were similar to those caused by a 3°C increase in water –1 –1 temperature. -
Assessment of the Flame Angelfish (Centropyge Loriculus) As a Model Species in Studies on Egg and Larval Quality in Marine Fishes Chatham K
The University of Maine DigitalCommons@UMaine Electronic Theses and Dissertations Fogler Library 8-2007 Assessment of the Flame Angelfish (Centropyge loriculus) as a Model Species in Studies on Egg and Larval Quality in Marine Fishes Chatham K. Callan Follow this and additional works at: http://digitalcommons.library.umaine.edu/etd Part of the Aquaculture and Fisheries Commons, and the Oceanography Commons Recommended Citation Callan, Chatham K., "Assessment of the Flame Angelfish (Centropyge loriculus) as a Model Species in Studies on Egg and Larval Quality in Marine Fishes" (2007). Electronic Theses and Dissertations. 126. http://digitalcommons.library.umaine.edu/etd/126 This Open-Access Dissertation is brought to you for free and open access by DigitalCommons@UMaine. It has been accepted for inclusion in Electronic Theses and Dissertations by an authorized administrator of DigitalCommons@UMaine. ASSESSMENT OF THE FLAME ANGELFISH (Centropyge loriculus) AS A MODEL SPECIES IN STUDIES ON EGG AND LARVAL QUALITY IN MARINE FISHES By Chatham K. Callan B.S. Fairleigh Dickinson University, 1997 M.S. University of Maine, 2000 A THESIS Submitted in Partial Fulfillment of the Requirements for the Degree of Doctor of Philosophy (in Marine Biology) The Graduate School The University of Maine August, 2007 Advisory Committee: David W. Townsend, Professor of Oceanography, Advisor Linda Kling, Associate Professor of Aquaculture and Fish Nutrition, Co-Advisor Denise Skonberg, Associate Professor of Food Science Mary Tyler, Professor of Biological Science Christopher Brown, Professor of Marine Science (Florida International University) LIBRARY RIGHTS STATEMENT In presenting this thesis in partial fulfillment of the requirements for an advanced degree at The University of Maine, I agree that the Library shall make it freely available for inspection. -
Suborder GOBIOIDEI ELEOTRIDAE Sleepers by E.O
click for previous page 1778 Bony Fishes Suborder GOBIOIDEI ELEOTRIDAE Sleepers by E.O. Murdy, National Science Foundation, Virginia, USA and D.F. Hoese, Australian Museum, Sydney, Australia iagnostic characters: Small to medium-sized (most do not exceed 20 cm, although Gobiomorus from Dthis area may reach 60 cm). Typically, body stout; head short and broad; snout blunt; gill membranes broadly joined to isthmus. Teeth usually small, conical and in several rows in jaws. Six branchiostegal rays. Two separate dorsal fins, first dorsal fin with 6 or 7 weak spines, second dorsal fin with 1 weak spine followed by 6 to 12 soft rays; second dorsal fin and anal fin relatively short-based; origin of anal fin just posterior to vertical with origin of second dorsal fin; terminal ray of second dorsal and anal fins divided to its base (but counted as a single element);anal fin with 1 weak spine followed by 6 to 12 soft rays;caudal fin broad and rounded, compris- ing 15 or 17 segmented rays; pectoral fin broad with 14 to 25 soft rays; pelvic fin long with 1 spine and 5 soft rays.Pelvic fins separate and not connected by a membrane.Scales large and either cycloid or ctenoid.No lateral line on body. Head typically scaled, scales being either cycloid or ctenoid with a series of sensory ca- nals and pores as well as cutaneous papillae. Colour: not brightly coloured, most are light or dark brown or olive with some metallic glints. Habitat, biology, and fisheries: Typically occur in fresh or brackish waters, although some species are truly marine. -
Long-Term Cleaning Patterns of the Sharknose Goby (Elacatinus Evelynae)
Long-term cleaning patterns of the sharknose goby (Elacatinus evelynae) ANGOR UNIVERSITY Dunkley, Katie; Ellison, Amy; Mohammed, Ryan S.; van Oosterhout, Cock; Whittey, Kathryn E.; Perkins, Sarah E.; Cable, Jo Coral Reefs DOI: 10.1007/s00338-019-01778-9 PRIFYSGOL BANGOR / B Published: 01/04/2019 Publisher's PDF, also known as Version of record Cyswllt i'r cyhoeddiad / Link to publication Dyfyniad o'r fersiwn a gyhoeddwyd / Citation for published version (APA): Dunkley, K., Ellison, A., Mohammed, R. S., van Oosterhout, C., Whittey, K. E., Perkins, S. E., & Cable, J. (2019). Long-term cleaning patterns of the sharknose goby (Elacatinus evelynae). Coral Reefs, 38(2), 321-330. https://doi.org/10.1007/s00338-019-01778-9 Hawliau Cyffredinol / General rights Copyright and moral rights for the publications made accessible in the public portal are retained by the authors and/or other copyright owners and it is a condition of accessing publications that users recognise and abide by the legal requirements associated with these rights. • Users may download and print one copy of any publication from the public portal for the purpose of private study or research. • You may not further distribute the material or use it for any profit-making activity or commercial gain • You may freely distribute the URL identifying the publication in the public portal ? Take down policy If you believe that this document breaches copyright please contact us providing details, and we will remove access to the work immediately and investigate your claim. 29. Sep. 2021 Coral Reefs https://doi.org/10.1007/s00338-019-01778-9 REPORT Long-term cleaning patterns of the sharknose goby (Elacatinus evelynae) 1 1 2 3 Katie Dunkley • Amy R. -
Seamap Environmental and Biological Atlas of the Gulf of Mexico, 2017
environmental and biological atlas of the gulf of mexico 2017 gulf states marine fisheries commission number 284 february 2019 seamap SEAMAP ENVIRONMENTAL AND BIOLOGICAL ATLAS OF THE GULF OF MEXICO, 2017 Edited by Jeffrey K. Rester Gulf States Marine Fisheries Commission Manuscript Design and Layout Ashley P. Lott Gulf States Marine Fisheries Commission GULF STATES MARINE FISHERIES COMMISSION FEBRUARY 2019 NUMBER 284 This project was supported in part by the National Oceanic and Atmospheric Administration, National Marine Fisheries Service, under State/Federal Project Number NA16NMFS4350111. GULF STATES MARINE FISHERIES COMMISSION COMMISSIONERS ALABAMA Chris Blankenship John Roussel Alabama Department of Conservation 1221 Plains Port Hudson Road and Natural Resources Zachary, LA 70791 64 North Union Street Montgomery, AL 36130-1901 MISSISSIPPI Joe Spraggins, Executive Director Representative Steve McMillan Mississippi Department of Marine Resources P.O. Box 337 1141 Bayview Avenue Bay Minette, AL 36507 Biloxi, MS 39530 Chris Nelson TBA Bon Secour Fisheries, Inc. P.O. Box 60 Joe Gill, Jr. Bon Secour, AL 36511 Joe Gill Consulting, LLC 910 Desoto Street FLORIDA Ocean Springs, MS 39566-0535 Eric Sutton FL Fish and Wildlife Conservation Commission TEXAS 620 South Meridian Street Carter Smith, Executive Director Tallahassee, FL 32399-1600 Texas Parks and Wildlife Department 4200 Smith School Road Representative Jay Trumbull Austin, TX 78744 State of Florida House of Representatives 402 South Monroe Street Troy B. Williamson, II Tallahassee, FL 32399 P.O. Box 967 Corpus Christi, TX 78403 TBA Representative Wayne Faircloth LOUISIANA Texas House of Representatives Jack Montoucet, Secretary 2121 Market Street, Suite 205 LA Department of Wildlife and Fisheries Galveston, TX 77550 P.O. -
Fish Brain Development in a Changing Ocean
UNIVERSIDADE DE LISBOA FACULDADE DE CIÊNCIAS DEPARTAMENTO DE BIOLOGIA ANIMAL Fish Brain Development in a Changing Ocean Francisco José Ferraz de Oliveira Soeiro de Carvalho Mestrado em Ecologia Marinha Dissertação orientada por: Professor Doutor Rui Rosa Professor Doutor Ricardo Calado 2018 UNIVERSIDADE DE LISBOA FACULDADE DE CIÊNCIAS DEPARTAMENTO DE BIOLOGIA ANIMAL Fish Brain Development in a Changing Ocean Francisco José Ferraz de Oliveira Soeiro de Carvalho Mestrado em Ecologia Marinha Dissertação orientada por: Professor Doutor Rui Rosa Professor Doutor Ricardo Calado 2018 To the fish and the humans of sea and land Aos peixes e aos humanos do mar e da terra Acknowledgements To the fish and the personnel in the old Guia Lab, from my careful advisor Rui Rosa and my “almost advisor” José Ricardo Paula to the humorous cleaner, And all the colleagues and young researchers with whom countless living hours in and out were shared. To my co-advisor Ricardo Calado and to my reviewer and good friend Tiago Freire. To the scientific community, direct and indirectly contacted in meetings and readings; and to my friends, skipper mates and family, inland and abroad in the seas, I consider and thank you all. III Funding This study was funded by Portuguese national funds through FCT – Fundação para a Ciência e Tecnologia, I.P., within the project MUTUALCHANGE: Bio-ecological responses of marine cleaning mutualisms to climate change (PTDC/MAR-EST/5880/2014). IV Presentations in conferences This work was presented in the following scientific meetings: - XIII Congresso da Sociedade Portuguesa de Etologia, 2016 Carvalho, F.; Paula, J. R.; Lopes, A. -
Materials and Methods
View metadata, citation and similar papers at core.ac.uk brought to you by CORE provided by Woods Hole Open Access Server 1 2 3 Planktonic Larval Duration, Age and Growth of Ostorhinchus doederleini (Pisces: Apogonidae) on 4 the Southern Great Barrier Reef, Australia 5 6 M.J. Kingsford1* 7 M.D. Finn1† 8 M.D. O’Callaghan1 9 J. Atema2 10 G. Gerlach3 11 1 ARC Centre of Excellence for Coral Reef Studies, School of Marine and Tropical Biology, James 12 Cook University, Townsville, QLD, Australia 4811 13 14 2 University of Boston, and Woodshole Oceanographic Institute 15 3Carl von Ossietzky University of Oldenburg Carl von Ossietzky Str. 9-11, 26111 Oldenburg, Germany 16 *Corresponding Author. 17 Phone: +61 7 4781 4345 18 FAX: +61 7 4781 5511 19 E-mail: [email protected] 20 †Current address: School of Marine and Tropical Biology, James Cook University, Townsville, Qld 21 4811 Australia 22 Keywords: Apogonidae, otoliths, age, PLD, settlement, growth, mortality. 23 24 1 25 26 Abstract 27 Cardinalfishes (Apogonidae) are abundant on corals reefs, but there are few data on demography to 28 understand trophodynamics and population dynamics. Ostorhinchus doederleini is a small and abundant 29 apogonid on the Great Barrier Reef (GBR) and throughout the Western Pacific Ocean. We present key 30 demographic parameters for the entire life history from the southern GBR. Daily deposition of 31 increments in otoliths was validated. Fish had a Planktonic Larval Duration (PLD) of 16 to 26 days. 32 PLD was established from fish collected immediately prior to settlement as no settlement mark was 33 found.