Comparative Genomics and Transcriptomics to Analyze Fruiting Body Development in Filamentous Ascomycetes
Total Page:16
File Type:pdf, Size:1020Kb
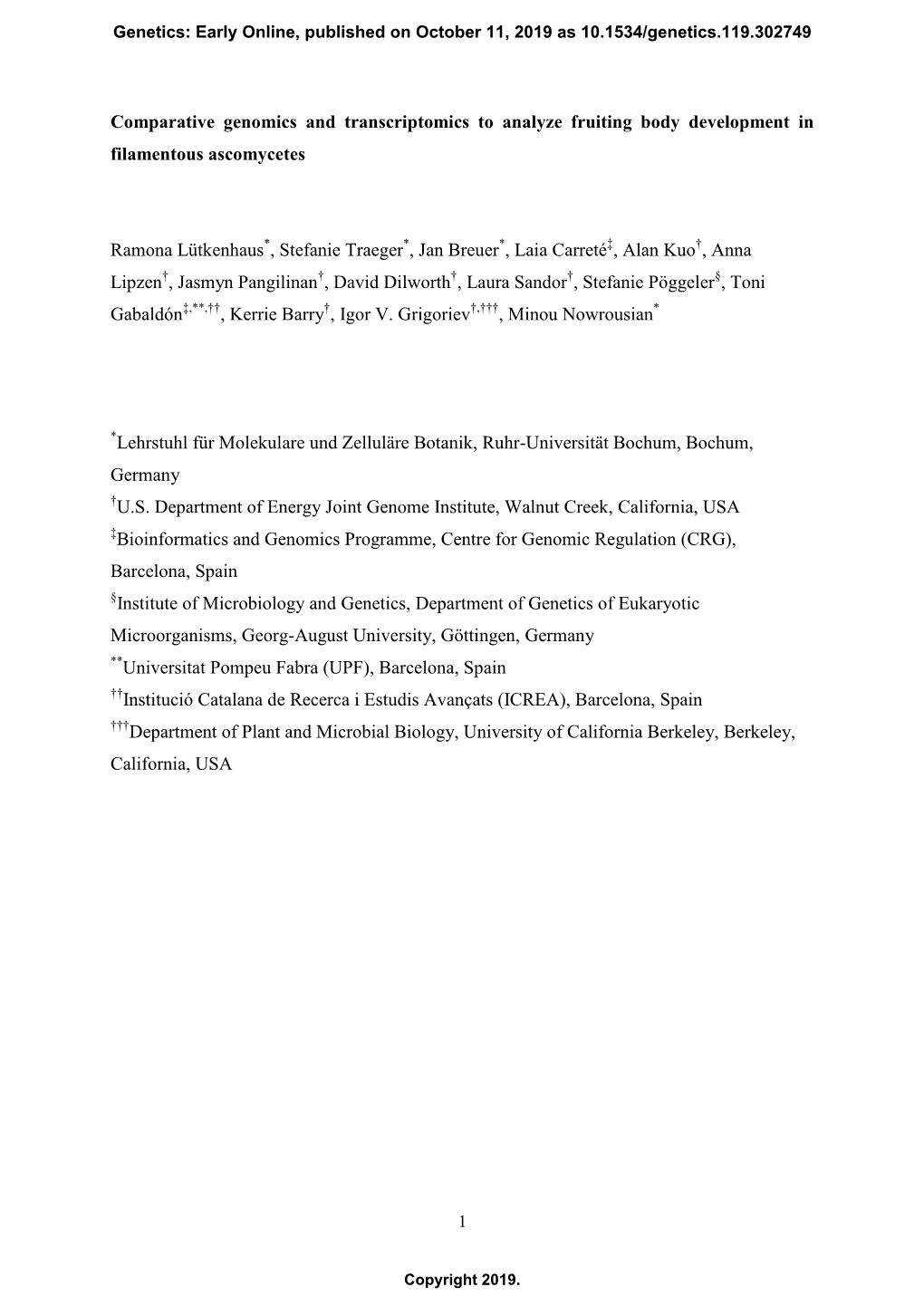
Load more
Recommended publications
-
How Many Fungi Make Sclerotia?
fungal ecology xxx (2014) 1e10 available at www.sciencedirect.com ScienceDirect journal homepage: www.elsevier.com/locate/funeco Short Communication How many fungi make sclerotia? Matthew E. SMITHa,*, Terry W. HENKELb, Jeffrey A. ROLLINSa aUniversity of Florida, Department of Plant Pathology, Gainesville, FL 32611-0680, USA bHumboldt State University of Florida, Department of Biological Sciences, Arcata, CA 95521, USA article info abstract Article history: Most fungi produce some type of durable microscopic structure such as a spore that is Received 25 April 2014 important for dispersal and/or survival under adverse conditions, but many species also Revision received 23 July 2014 produce dense aggregations of tissue called sclerotia. These structures help fungi to survive Accepted 28 July 2014 challenging conditions such as freezing, desiccation, microbial attack, or the absence of a Available online - host. During studies of hypogeous fungi we encountered morphologically distinct sclerotia Corresponding editor: in nature that were not linked with a known fungus. These observations suggested that Dr. Jean Lodge many unrelated fungi with diverse trophic modes may form sclerotia, but that these structures have been overlooked. To identify the phylogenetic affiliations and trophic Keywords: modes of sclerotium-forming fungi, we conducted a literature review and sequenced DNA Chemical defense from fresh sclerotium collections. We found that sclerotium-forming fungi are ecologically Ectomycorrhizal diverse and phylogenetically dispersed among 85 genera in 20 orders of Dikarya, suggesting Plant pathogens that the ability to form sclerotia probably evolved 14 different times in fungi. Saprotrophic ª 2014 Elsevier Ltd and The British Mycological Society. All rights reserved. Sclerotium Fungi are among the most diverse lineages of eukaryotes with features such as a hyphal thallus, non-flagellated cells, and an estimated 5.1 million species (Blackwell, 2011). -
Phylogenetic Investigations of Sordariaceae Based on Multiple Gene Sequences and Morphology
mycological research 110 (2006) 137– 150 available at www.sciencedirect.com journal homepage: www.elsevier.com/locate/mycres Phylogenetic investigations of Sordariaceae based on multiple gene sequences and morphology Lei CAI*, Rajesh JEEWON, Kevin D. HYDE Centre for Research in Fungal Diversity, Department of Ecology & Biodiversity, The University of Hong Kong, Pokfulam Road, Hong Kong SAR, PR China article info abstract Article history: The family Sordariaceae incorporates a number of fungi that are excellent model organisms Received 10 May 2005 for various biological, biochemical, ecological, genetic and evolutionary studies. To deter- Received in revised form mine the evolutionary relationships within this group and their respective phylogenetic 19 August 2005 placements, multiple-gene sequences (partial nuclear 28S ribosomal DNA, nuclear ITS ribo- Accepted 29 September 2005 somal DNA and partial nuclear b-tubulin) were analysed using maximum parsimony and Corresponding Editor: H. Thorsten Bayesian analyses. Analyses of different gene datasets were performed individually and Lumbsch then combined to generate phylogenies. We report that Sordariaceae, with the exclusion Apodus and Diplogelasinospora, is a monophyletic group. Apodus and Diplogelasinospora are Keywords: related to Lasiosphaeriaceae. Multiple gene analyses suggest that the spore sheath is not Ascomycota a phylogenetically significant character to segregate Asordaria from Sordaria. Smooth- Gelasinospora spored Sordaria species (including so-called Asordaria species) constitute a natural group. Neurospora Asordaria is therefore congeneric with Sordaria. Anixiella species nested among Gelasinospora Sordaria species, providing further evidence that non-ostiolate ascomata have evolved from ostio- late ascomata on several independent occasions. This study agrees with previous studies that show heterothallic Neurospora species to be monophyletic, but that homothallic ones may have a multiple origins. -
Preliminary Classification of Leotiomycetes
Mycosphere 10(1): 310–489 (2019) www.mycosphere.org ISSN 2077 7019 Article Doi 10.5943/mycosphere/10/1/7 Preliminary classification of Leotiomycetes Ekanayaka AH1,2, Hyde KD1,2, Gentekaki E2,3, McKenzie EHC4, Zhao Q1,*, Bulgakov TS5, Camporesi E6,7 1Key Laboratory for Plant Diversity and Biogeography of East Asia, Kunming Institute of Botany, Chinese Academy of Sciences, Kunming 650201, Yunnan, China 2Center of Excellence in Fungal Research, Mae Fah Luang University, Chiang Rai, 57100, Thailand 3School of Science, Mae Fah Luang University, Chiang Rai, 57100, Thailand 4Landcare Research Manaaki Whenua, Private Bag 92170, Auckland, New Zealand 5Russian Research Institute of Floriculture and Subtropical Crops, 2/28 Yana Fabritsiusa Street, Sochi 354002, Krasnodar region, Russia 6A.M.B. Gruppo Micologico Forlivese “Antonio Cicognani”, Via Roma 18, Forlì, Italy. 7A.M.B. Circolo Micologico “Giovanni Carini”, C.P. 314 Brescia, Italy. Ekanayaka AH, Hyde KD, Gentekaki E, McKenzie EHC, Zhao Q, Bulgakov TS, Camporesi E 2019 – Preliminary classification of Leotiomycetes. Mycosphere 10(1), 310–489, Doi 10.5943/mycosphere/10/1/7 Abstract Leotiomycetes is regarded as the inoperculate class of discomycetes within the phylum Ascomycota. Taxa are mainly characterized by asci with a simple pore blueing in Melzer’s reagent, although some taxa have lost this character. The monophyly of this class has been verified in several recent molecular studies. However, circumscription of the orders, families and generic level delimitation are still unsettled. This paper provides a modified backbone tree for the class Leotiomycetes based on phylogenetic analysis of combined ITS, LSU, SSU, TEF, and RPB2 loci. In the phylogenetic analysis, Leotiomycetes separates into 19 clades, which can be recognized as orders and order-level clades. -
New Records of Aspergillus Allahabadii and Penicillium Sizovae
MYCOBIOLOGY 2018, VOL. 46, NO. 4, 328–340 https://doi.org/10.1080/12298093.2018.1550169 RESEARCH ARTICLE Four New Records of Ascomycete Species from Korea Thuong T. T. Nguyen, Monmi Pangging, Seo Hee Lee and Hyang Burm Lee Division of Food Technology, Biotechnology and Agrochemistry, College of Agriculture & Life Sciences, Chonnam National University, Gwangju, Korea ABSTRACT ARTICLE HISTORY While evaluating fungal diversity in freshwater, grasshopper feces, and soil collected at Received 3 July 2018 Dokdo Island in Korea, four fungal strains designated CNUFC-DDS14-1, CNUFC-GHD05-1, Revised 27 September 2018 CNUFC-DDS47-1, and CNUFC-NDR5-2 were isolated. Based on combination studies using Accepted 28 October 2018 phylogenies and morphological characteristics, the isolates were confirmed as Ascodesmis KEYWORDS sphaerospora, Chaetomella raphigera, Gibellulopsis nigrescens, and Myrmecridium schulzeri, Ascomycetes; fecal; respectively. This is the first records of these four species from Korea. freshwater; fungal diversity; soil 1. Introduction Paraphoma, Penicillium, Plectosphaerella, and Stemphylium [7–11]. However, comparatively few Fungi represent an integral part of the biomass of any species of fungi have been described [8–10]. natural environment including soils. In soils, they act Freshwater nourishes diverse habitats for fungi, as agents governing soil carbon cycling, plant nutri- such as fallen leaves, plant litter, decaying wood, tion, and pathology. Many fungal species also adapt to aquatic plants and insects, and soils. Little -
The Phylogeny of Plant and Animal Pathogens in the Ascomycota
Physiological and Molecular Plant Pathology (2001) 59, 165±187 doi:10.1006/pmpp.2001.0355, available online at http://www.idealibrary.com on MINI-REVIEW The phylogeny of plant and animal pathogens in the Ascomycota MARY L. BERBEE* Department of Botany, University of British Columbia, 6270 University Blvd, Vancouver, BC V6T 1Z4, Canada (Accepted for publication August 2001) What makes a fungus pathogenic? In this review, phylogenetic inference is used to speculate on the evolution of plant and animal pathogens in the fungal Phylum Ascomycota. A phylogeny is presented using 297 18S ribosomal DNA sequences from GenBank and it is shown that most known plant pathogens are concentrated in four classes in the Ascomycota. Animal pathogens are also concentrated, but in two ascomycete classes that contain few, if any, plant pathogens. Rather than appearing as a constant character of a class, the ability to cause disease in plants and animals was gained and lost repeatedly. The genes that code for some traits involved in pathogenicity or virulence have been cloned and characterized, and so the evolutionary relationships of a few of the genes for enzymes and toxins known to play roles in diseases were explored. In general, these genes are too narrowly distributed and too recent in origin to explain the broad patterns of origin of pathogens. Co-evolution could potentially be part of an explanation for phylogenetic patterns of pathogenesis. Robust phylogenies not only of the fungi, but also of host plants and animals are becoming available, allowing for critical analysis of the nature of co-evolutionary warfare. Host animals, particularly human hosts have had little obvious eect on fungal evolution and most cases of fungal disease in humans appear to represent an evolutionary dead end for the fungus. -
Henry Dissing, 31. March 1931 – 10. December 2009
Henry Dissing, 31. March 1931 – 10. December 2009 Thomas LÆSSØE Department of Biology, University of Copenhagen Universitetsparken 15 DK-2100 Copenhagen Ø [email protected] Ascomycete.org, 2 (4) : 3-6. Summary: Biography of Henry Dissing, Danish mycologist, specialist of Pezizales, died Février 2011 in December 2009. Keywords: Tribute, Danish mycologist, University of Copenhagen, Ascomycota. Résumé : biographie d’Henry Dissing, mycologue danois, spécialiste des Pezizales, dé- cédé en décembre 2009. Mots-clés : hommage, mycologue danois, université de Copenhague, Ascomycota. Henry was born in Jutland, in a small village, where he was association was with Sigmund Sivertsen in Norway. Throu- expected to follow in his father’s footsteps as a potter. He ghout, he trained Master students in all sorts of mycological chose a completely different career but did support his early topics, and one of them, Karen Hansen, continues his work education by working at the royal porcelain factory in Co- on the Pezizales (from Stockholm). Others are employed in penhagen. After that, he studied at Copenhagen University the biotechnological industry or teach at high school. A long where he started his biology studies in 1960. He very soon lasting teaching effort was the mycological field courses held became interested in fungi and quickly became part of the from 1965-2009 at the Kristiansminde Field Centre, where group around Morten Lange at the newly established “Insti- Henry participated in most courses until retirement, and tut for Sporeplanter”, where Lise Hansen was another core more than one thousand students got their mycological field member. Henry became the ascomycote person and Morten training during this period, including a lot of Norwegian stu- dealt with agarics and also collaborated with Henry on “Gas- dents. -
Fungal Cannons: Explosive Spore Discharge in the Ascomycota Frances Trail
MINIREVIEW Fungal cannons: explosive spore discharge in the Ascomycota Frances Trail Department of Plant Biology and Department of Plant Pathology, Michigan State University, East Lansing, MI, USA Correspondence: Frances Trail, Department Abstract Downloaded from https://academic.oup.com/femsle/article/276/1/12/593867 by guest on 24 September 2021 of Plant Biology, Michigan State University, East Lansing, MI 48824, USA. Tel.: 11 517 The ascomycetous fungi produce prodigious amounts of spores through both 432 2939; fax: 11 517 353 1926; asexual and sexual reproduction. Their sexual spores (ascospores) develop within e-mail: [email protected] tubular sacs called asci that act as small water cannons and expel the spores into the air. Dispersal of spores by forcible discharge is important for dissemination of Received 15 June 2007; revised 28 July 2007; many fungal plant diseases and for the dispersal of many saprophytic fungi. The accepted 30 July 2007. mechanism has long been thought to be driven by turgor pressure within the First published online 3 September 2007. extending ascus; however, relatively little genetic and physiological work has been carried out on the mechanism. Recent studies have measured the pressures within DOI:10.1111/j.1574-6968.2007.00900.x the ascus and quantified the components of the ascus epiplasmic fluid that contribute to the osmotic potential. Few species have been examined in detail, Editor: Richard Staples but the results indicate diversity in ascus function that reflects ascus size, fruiting Keywords body type, and the niche of the particular species. ascus; ascospore; turgor pressure; perithecium; apothecium. 2 and 3). Each subphylum contains members that forcibly Introduction discharge their spores. -
Orbilia Ultrastructure, Character Evolution and Phylogeny of Pezizomycotina
Mycologia, 104(2), 2012, pp. 462–476. DOI: 10.3852/11-213 # 2012 by The Mycological Society of America, Lawrence, KS 66044-8897 Orbilia ultrastructure, character evolution and phylogeny of Pezizomycotina T.K. Arun Kumar1 INTRODUCTION Department of Plant Biology, University of Minnesota, St Paul, Minnesota 55108 Ascomycota is a monophyletic phylum (Lutzoni et al. 2004, James et al. 2006, Spatafora et al. 2006, Hibbett Rosanne Healy et al. 2007) comprising three subphyla, Taphrinomy- Department of Plant Biology, University of Minnesota, cotina, Saccharomycotina and Pezizomycotina (Su- St Paul, Minnesota 55108 giyama et al. 2006, Hibbett et al. 2007). Taphrinomy- Joseph W. Spatafora cotina, according to the current classification (Hibbett Department of Botany and Plant Pathology, Oregon et al. 2007), consists of four classes, Neolectomycetes, State University, Corvallis, Oregon 97331 Pneumocystidiomycetes, Schizosaccharomycetes, Ta- phrinomycetes, and an unplaced genus, Saitoella, Meredith Blackwell whose members are ecologically and morphologically Department of Biological Sciences, Louisiana State University, Baton Rouge, Louisiana 70803 highly diverse (Sugiyama et al. 2006). Soil Clone Group 1, poorly known from geographically wide- David J. McLaughlin spread environmental samples and a single culture, Department of Plant Biology, University of Minnesota, was suggested as a fourth subphylum (Porter et al. St Paul, Minnesota 55108 2008). More recently however the group has been described as a new class of Taphrinomycotina, Archae- orhizomycetes (Rosling et al. 2011), based primarily on Abstract: Molecular phylogenetic analyses indicate information from rRNA sequences. The mode of that the monophyletic classes Orbiliomycetes and sexual reproduction in Taphrinomycotina is ascogen- Pezizomycetes are among the earliest diverging ous without the formation of ascogenous hyphae, and branches of Pezizomycotina, the largest subphylum except for the enigmatic, apothecium-producing of the Ascomycota. -
Coprophilous Fungal Community of Wild Rabbit in a Park of a Hospital (Chile): a Taxonomic Approach
Boletín Micológico Vol. 21 : 1 - 17 2006 COPROPHILOUS FUNGAL COMMUNITY OF WILD RABBIT IN A PARK OF A HOSPITAL (CHILE): A TAXONOMIC APPROACH (Comunidades fúngicas coprófilas de conejos silvestres en un parque de un Hospital (Chile): un enfoque taxonómico) Eduardo Piontelli, L, Rodrigo Cruz, C & M. Alicia Toro .S.M. Universidad de Valparaíso, Escuela de Medicina Cátedra de micología, Casilla 92 V Valparaíso, Chile. e-mail <eduardo.piontelli@ uv.cl > Key words: Coprophilous microfungi,wild rabbit, hospital zone, Chile. Palabras clave: Microhongos coprófilos, conejos silvestres, zona de hospital, Chile ABSTRACT RESUMEN During year 2005-through 2006 a study on copro- Durante los años 2005-2006 se efectuó un estudio philous fungal communities present in wild rabbit dung de las comunidades fúngicas coprófilos en excementos de was carried out in the park of a regional hospital (V conejos silvestres en un parque de un hospital regional Region, Chile), 21 samples in seven months under two (V Región, Chile), colectándose 21 muestras en 7 meses seasonable periods (cold and warm) being collected. en 2 períodos estacionales (fríos y cálidos). Un total de Sixty species and 44 genera as a total were recorded in 60 especies y 44 géneros fueron detectados en el período the sampling period, 46 species in warm periods and 39 de muestreo, 46 especies en los períodos cálidos y 39 en in the cold ones. Major groups were arranged as follows: los fríos. La distribución de los grandes grupos fue: Zygomycota (11,6 %), Ascomycota (50 %), associated Zygomycota(11,6 %), Ascomycota (50 %), géneros mitos- mitosporic genera (36,8 %) and Basidiomycota (1,6 %). -
A Higher-Level Phylogenetic Classification of the Fungi
mycological research 111 (2007) 509–547 available at www.sciencedirect.com journal homepage: www.elsevier.com/locate/mycres A higher-level phylogenetic classification of the Fungi David S. HIBBETTa,*, Manfred BINDERa, Joseph F. BISCHOFFb, Meredith BLACKWELLc, Paul F. CANNONd, Ove E. ERIKSSONe, Sabine HUHNDORFf, Timothy JAMESg, Paul M. KIRKd, Robert LU¨ CKINGf, H. THORSTEN LUMBSCHf, Franc¸ois LUTZONIg, P. Brandon MATHENYa, David J. MCLAUGHLINh, Martha J. POWELLi, Scott REDHEAD j, Conrad L. SCHOCHk, Joseph W. SPATAFORAk, Joost A. STALPERSl, Rytas VILGALYSg, M. Catherine AIMEm, Andre´ APTROOTn, Robert BAUERo, Dominik BEGEROWp, Gerald L. BENNYq, Lisa A. CASTLEBURYm, Pedro W. CROUSl, Yu-Cheng DAIr, Walter GAMSl, David M. GEISERs, Gareth W. GRIFFITHt,Ce´cile GUEIDANg, David L. HAWKSWORTHu, Geir HESTMARKv, Kentaro HOSAKAw, Richard A. HUMBERx, Kevin D. HYDEy, Joseph E. IRONSIDEt, Urmas KO˜ LJALGz, Cletus P. KURTZMANaa, Karl-Henrik LARSSONab, Robert LICHTWARDTac, Joyce LONGCOREad, Jolanta MIA˛ DLIKOWSKAg, Andrew MILLERae, Jean-Marc MONCALVOaf, Sharon MOZLEY-STANDRIDGEag, Franz OBERWINKLERo, Erast PARMASTOah, Vale´rie REEBg, Jack D. ROGERSai, Claude ROUXaj, Leif RYVARDENak, Jose´ Paulo SAMPAIOal, Arthur SCHU¨ ßLERam, Junta SUGIYAMAan, R. Greg THORNao, Leif TIBELLap, Wendy A. UNTEREINERaq, Christopher WALKERar, Zheng WANGa, Alex WEIRas, Michael WEISSo, Merlin M. WHITEat, Katarina WINKAe, Yi-Jian YAOau, Ning ZHANGav aBiology Department, Clark University, Worcester, MA 01610, USA bNational Library of Medicine, National Center for Biotechnology Information, -
The Classification of Lower Organisms
The Classification of Lower Organisms Ernst Hkinrich Haickei, in 1874 From Rolschc (1906). By permission of Macrae Smith Company. C f3 The Classification of LOWER ORGANISMS By HERBERT FAULKNER COPELAND \ PACIFIC ^.,^,kfi^..^ BOOKS PALO ALTO, CALIFORNIA Copyright 1956 by Herbert F. Copeland Library of Congress Catalog Card Number 56-7944 Published by PACIFIC BOOKS Palo Alto, California Printed and bound in the United States of America CONTENTS Chapter Page I. Introduction 1 II. An Essay on Nomenclature 6 III. Kingdom Mychota 12 Phylum Archezoa 17 Class 1. Schizophyta 18 Order 1. Schizosporea 18 Order 2. Actinomycetalea 24 Order 3. Caulobacterialea 25 Class 2. Myxoschizomycetes 27 Order 1. Myxobactralea 27 Order 2. Spirochaetalea 28 Class 3. Archiplastidea 29 Order 1. Rhodobacteria 31 Order 2. Sphaerotilalea 33 Order 3. Coccogonea 33 Order 4. Gloiophycea 33 IV. Kingdom Protoctista 37 V. Phylum Rhodophyta 40 Class 1. Bangialea 41 Order Bangiacea 41 Class 2. Heterocarpea 44 Order 1. Cryptospermea 47 Order 2. Sphaerococcoidea 47 Order 3. Gelidialea 49 Order 4. Furccllariea 50 Order 5. Coeloblastea 51 Order 6. Floridea 51 VI. Phylum Phaeophyta 53 Class 1. Heterokonta 55 Order 1. Ochromonadalea 57 Order 2. Silicoflagellata 61 Order 3. Vaucheriacea 63 Order 4. Choanoflagellata 67 Order 5. Hyphochytrialea 69 Class 2. Bacillariacea 69 Order 1. Disciformia 73 Order 2. Diatomea 74 Class 3. Oomycetes 76 Order 1. Saprolegnina 77 Order 2. Peronosporina 80 Order 3. Lagenidialea 81 Class 4. Melanophycea 82 Order 1 . Phaeozoosporea 86 Order 2. Sphacelarialea 86 Order 3. Dictyotea 86 Order 4. Sporochnoidea 87 V ly Chapter Page Orders. Cutlerialea 88 Order 6. -
Ascomyceteorg 02-04 Ascomyceteorg
The genus Ascodesmis (Pezizales) in Norway Roy KRISTIANSEN PO Box 32 NO-1650 Sellebakk [email protected] Ascomycete.org, 2 (4) : 65-69. Summary: This report presents the four species of Ascodesmis (Ascomycota, Pezi- Février 2011 zales) recorded in Norway, and an updated map of their distribution. All are copro- philous, and occur on dung of both carnivorous and herbivorous animals. The species are illustrated by scanning electronmicrography of their ascospores, which is the main distinguishing character at species level. Systematics are briefly discussed, and As- codesmis is genetically close to the genus Eleutherascus. Keywords: Ascomycota, Pezizales, Ascodesmidaceae, Ascodesmis, Norway, distribu- tion. Ascodesmis Tiegh. (Ascodesmidaceae J. Schröt.), is one of and the differentiation of the primary and secondary ascos- the smallest genera in the Pezizales, comprising six species pore wall is equal in every detail in both genera (BRUMMELEN, world-wide, and all are strictly coprophilous. They are rarely 1989). This confirms that Eleutherascus is genetically clo- collected, but probably overlooked or have escaped atten- sest to Ascodesmis and that it should be placed in the As- tion because of their size, which hardly exceeds 0.5 mm in codesmidaceae. The similarity was later demonstrated by diameter, or they are really rare. The ascomata are rather phylogenetic studies, and which indicated also that there is simple in anatomy and consist of a bunch of asci with a li- a close relationship between Ascodesmidaceae and Pyro- mited number of colourless paraphyses, and almost no ex- nemataceae (PERRY et al., 2007), and confirms the close re- cipulum, with only a few basal cells.