Determination of Laterality in the Rabbit Embryo: Studies on Ciliation and Asymmetric Signal Transfer
Total Page:16
File Type:pdf, Size:1020Kb
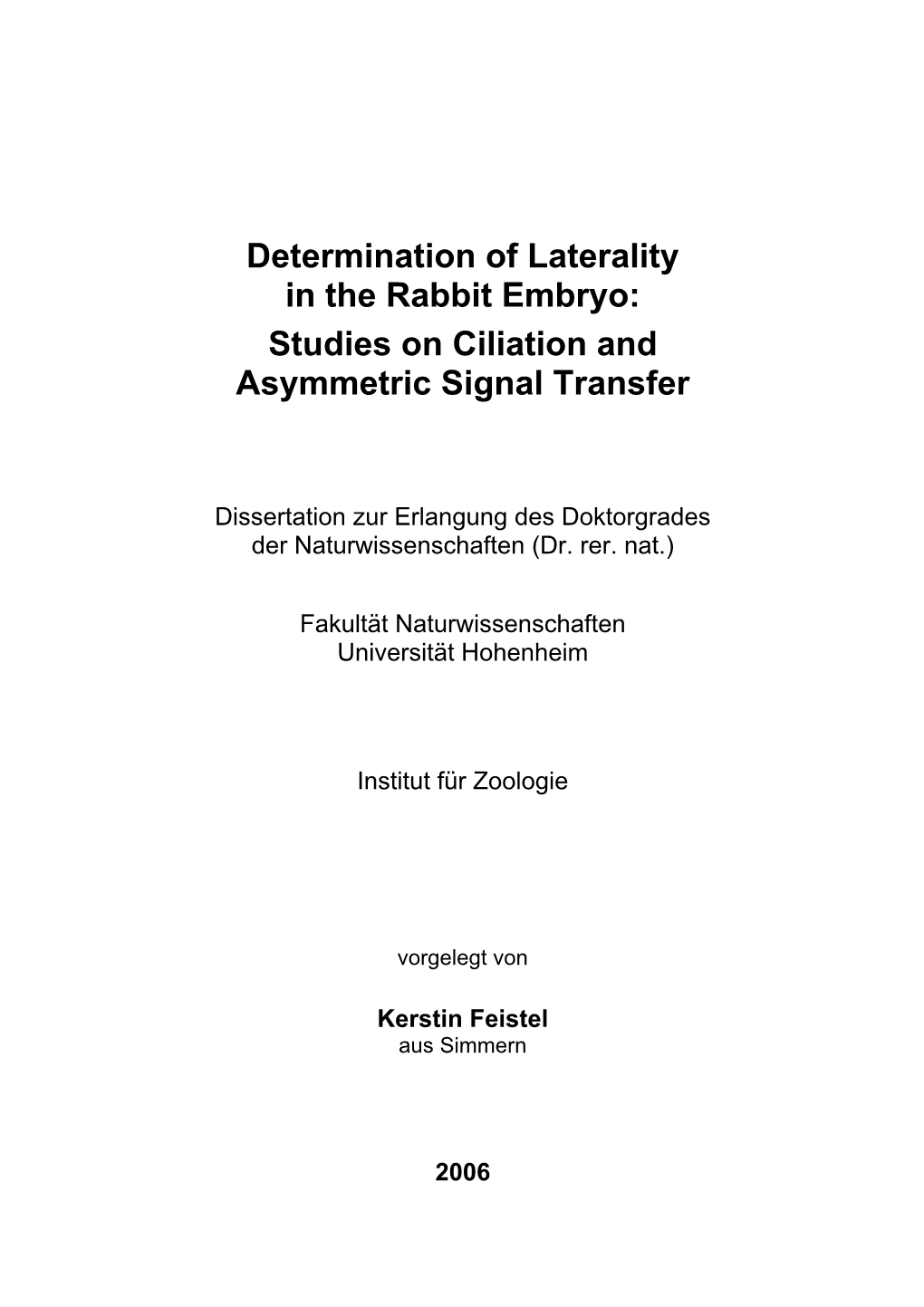
Load more
Recommended publications
-
From Bipotent Neuromesodermal Progenitors to Neural-Mesodermal Interactions During Embryonic Development
International Journal of Molecular Sciences Review From Bipotent Neuromesodermal Progenitors to Neural-Mesodermal Interactions during Embryonic Development Nitza Kahane and Chaya Kalcheim * Department of Medical Neurobiology, Institute of Medical Research Israel-Canada (IMRIC) and the Edmond and Lily Safra Center for Brain Sciences (ELSC), Hebrew University of Jerusalem-Hadassah Medical School, P.O. Box 12272, Jerusalem 9112102, Israel; [email protected] * Correspondence: [email protected] Abstract: To ensure the formation of a properly patterned embryo, multiple processes must operate harmoniously at sequential phases of development. This is implemented by mutual interactions between cells and tissues that together regulate the segregation and specification of cells, their growth and morphogenesis. The formation of the spinal cord and paraxial mesoderm derivatives exquisitely illustrate these processes. Following early gastrulation, while the vertebrate body elongates, a pop- ulation of bipotent neuromesodermal progenitors resident in the posterior region of the embryo generate both neural and mesodermal lineages. At later stages, the somitic mesoderm regulates aspects of neural patterning and differentiation of both central and peripheral neural progenitors. Reciprocally, neural precursors influence the paraxial mesoderm to regulate somite-derived myogen- esis and additional processes by distinct mechanisms. Central to this crosstalk is the activity of the axial notochord, which, via sonic hedgehog signaling, plays pivotal roles in neural, skeletal muscle and cartilage ontogeny. Here, we discuss the cellular and molecular basis underlying this complex Citation: Kahane, N.; Kalcheim, C. developmental plan, with a focus on the logic of sonic hedgehog activities in the coordination of the From Bipotent Neuromesodermal Progenitors to Neural-Mesodermal neural-mesodermal axis. -
Genes for Extracellular Matrix-Degrading Metalloproteinases and Their Inhibitor, TIMP, Are Expressed During Early Mammalian Development
Downloaded from genesdev.cshlp.org on September 23, 2021 - Published by Cold Spring Harbor Laboratory Press Genes for extracellular matrix-degrading metalloproteinases and their inhibitor, TIMP, are expressed during early mammalian development Carol A. Brenner, 1 Richard R. Adler, 2 Daniel A. Rappolee, Roger A. Pedersen, and Zena Werb 3 Laboratory of Radiobiology and Environmental Health, and Department of Anatomy, University of California, San Francisco, California 94143-0750 USA Extracellular matrix (ECM) remodeling accompanies cell migration, cell--cell interactions, embryo expansion, uterine implantation, and tissue invasion during mammalian embryogenesis. We have found that mouse embryos secrete functional ECM-degrading metalloproteinases, including collagenase and stromelysin, that are inhibitable by the tissue inhibitor of metalloproteinases (TIMP) and that are regulated during peri-implantation development and endoderm differentiation, mRNA transcripts for collagenase, stromelysin, and TIMP were detected as maternal transcripts in the unfertilized egg, were present at the zygote and cleavage stages, and increased at the blastocyst stage and with endoderm differentiation. These data suggest that metalloproteinases function in cell-ECM interactions during growth, development, and implantation of mammalian embryos. [Key Words: Collagenase; stromelysin; polymerase chain reaction; tissue inhibitor of metalloproteinases; maternal mRNA; endoderm differentiation] January 31, 1989; revised version accepted March 15, 1989. The extracellular matrix (ECM) forms a substrate for cell 1988}. The activity of these proteinases is regulated by attachment and migration and directs cell form and the tissue inhibitor of metalloproteinases {TIMPI function through cell-ECM interactions. ECM macro- (Murphy et al. 1985; Herron et al. 1986a, b; Gavrilovic et molecules first appear in the mammalian embryo in the al. -
Analysis of Mouse Embryonic Patterning and Morphogenesis By
Analysis of mouse embryonic patterning and INAUGURAL ARTICLE morphogenesis by forward genetics Marı´a J. Garcı´a-Garcı´a*,Jonathan T. Eggenschwiler*†, Tamara Caspary*‡, Heather L. Alcorn*, Michael R. Wyler*, Danwei Huangfu*§, Andrew S. Rakeman*¶, Jeffrey D. Lee*, Evan H. Feinberg*ʈ, John R. Timmer*,**, and Kathryn V. Anderson*†† *Developmental Biology Program, Sloan–Kettering Institute, 1275 York Avenue, New York, NY 10021; §Neuroscience Program and ¶Molecular and Cell Biology Program, Weill Graduate School of Medical Sciences, Cornell University, 445 East 69th Street, New York, NY 10021; and ʈWeill Graduate School of Medical Sciences at Cornell University͞The Rockefeller University͞Sloan–Kettering Institute Tri-Institutional M.D.-Ph.D. Program, 1300 York Avenue, New York, NY 10021 This contribution is part of the special series of Inaugural Articles by members of the National Academy of Sciences elected on April 20, 2004. Contributed by Kathryn V. Anderson, February 8, 2005 Many aspects of the genetic control of mammalian embryogenesis proaches in other laboratories that focused on later stages of cannot be extrapolated from other animals. Taking a forward embryonic and fetal development in the mouse have also genetic approach, we have induced recessive mutations by treat- identified novel mutations successfully (9–11). With the avail- ment of mice with ethylnitrosourea and have identified 43 muta- ability of the mouse genome sequence, it has become straight- tions that affect early morphogenesis and patterning, including 38 forward to identify the genes responsible for the mutant genes that have not been studied previously. The molecular lesions phenotypes. responsible for 14 mutations were identified, including mutations Here, we describe the identification of 43 recessive mutations in nine genes that had not been characterized previously. -
Title: Assembly of Embryonic and Extra-Embryonic Stem Cells to Mimic Embryogenesis in Vitro
Title: Assembly of embryonic and extra-embryonic stem cells to mimic embryogenesis in vitro Authors: Sarah Ellys Harrison1,3, Berna Sozen1,2,3, Neophytos Christodoulou1, Christos Kyprianou1 and Magdalena Zernicka-Goetz1* Affiliations: 1 Mammalian Embryo and Stem Cell Group, University of Cambridge, Department of Physiology, Development and Neuroscience; Downing Street, Cambridge, CB2 3DY, UK 2 Department of Histology and Embryology, Faculty of Medicine, Akdeniz University, Antalya, 07070, Turkey 3 equal contribution *corresponding author Abstract: Mammalian embryogenesis requires intricate interactions between embryonic and extra- embryonic tissues to orchestrate and coordinate morphogenesis with changes in developmental potential. Here, we combine mouse embryonic stem cells (ESCs) and extra-embryonic trophoblast stem cells (TSCs) in a 3D-scaffold to generate structures whose morphogenesis is remarkably similar to natural embryos. By using genetically-modified stem cells and specific inhibitors, we show embryogenesis of ESC- and TSC-derived embryos, ETS-embryos, depends on crosstalk involving Nodal signaling. When ETS-embryos develop, they spontaneously initiate expression of mesoderm and primordial germ cell markers asymmetrically on the embryonic and extra-embryonic border, in response to Wnt and BMP signaling. Our study demonstrates the ability of distinct stem cell types to self-assemble in vitro to generate embryos whose morphogenesis, architecture, and constituent cell-types resemble natural embryos. 2 Main Text: Early mammalian development requires the formation of embryonic and extra-embryonic tissues and their cooperative interactions. As a result of this partnership, the embryonic tissue, epiblast, will become patterned to generate cells of the future organism. Concomitantly, the extra- embryonic tissues, the trophectoderm and primitive endoderm, will form the placenta and the yolk sac. -
Analysis of Human Embryos from Zygote to Blastocyst Reveals Distinct Gene Expression Patterns Relative to the Mouse
Developmental Biology 375 (2013) 54–64 Contents lists available at SciVerse ScienceDirect Developmental Biology journal homepage: www.elsevier.com/locate/developmentalbiology Analysis of human embryos from zygote to blastocyst reveals distinct gene expression patterns relative to the mouse Kathy K. Niakan a,b,n, Kevin Eggan a,nn a The Howard Hughes Medical Institute, Harvard Stem Cell Institute and the Department of Stem Cell and Regenerative Biology, Harvard University, 7 Divinity Avenue, Cambridge, MA 02138, USA b Centre for Trophoblast Research, Department of Physiology, Development and Neuroscience, Anne McLaren Laboratory for Regenerative Medicine, University of Cambridge, West Forvie Building, Robinson Way, Cambridge CB2 0SZ, UK article info abstract Article history: Early mammalian embryogenesis is controlled by mechanisms governing the balance between Received 21 July 2012 pluripotency and differentiation. The expression of early lineage-specific genes can vary significantly Received in revised form between species, with implications for developmental control and stem cell derivation. However, the 29 November 2012 mechanisms involved in patterning the human embryo are still unclear. We analyzed the appearance Accepted 11 December 2012 and localization of lineage-specific transcription factors in staged preimplantation human embryos Available online 19 December 2012 from the zygote until the blastocyst. We observed that the pluripotency-associated transcription Keywords: factor OCT4 was initially expressed in 8-cell embryos at 3 days post-fertilization (dpf), and restricted to Human embryo the inner cell mass (ICM) in 128–256 cell blastocysts (6 dpf), approximately 2 days later than the Human embryonic stem cell derivation mouse. The trophectoderm (TE)-associated transcription factor CDX2 was upregulated in 5 dpf Primitive endoderm blastocysts and initially coincident with OCT4, indicating a lag in CDX2 initiation in the TE lineage, Epiblast progenitor Trophectoderm relative to the mouse. -
Gastrulation in Mus Musculus (Common House Mouse) [1]
Published on The Embryo Project Encyclopedia (https://embryo.asu.edu) Gastrulation in Mus musculus (common house mouse) [1] By: Wolter, Justin M. Keywords: Model organisms [2] Mice [3] Gastrulation [4] Germ layers [5] As mice embryos develop, they undergo a stage of development calledg astrulation [7]. The hallmark of vertebrate gastrulation [7] is the reorganization of the inner cell mass [8] (ICM) into the three germ layers [9]: ectoderm [10], mesoderm [11], and endoderm [12]. Mammalian embryogenesis [13] occurs within organisms; therefore, gastrulation [7] was originally described in species with easily observable embryos. For example, the African clawed frog [14] (Xenopus laevis [15]) is a widely used organism to study gastrulation [7] because the large embryos develop inside a translucent membrane. Domestic chickens G( allus gallus) provided researchers another early model to study gastrulation [7] because researchers could open the egg [16] during development to look inside. Despite the challenges associated with studying mammalian gastrulation [7], the common house mouse [17] (Mus musculus) has helped to shed light on the unique adaptations associated with mammalian development. Gastrulation in the mouse [6] begins shortly after a blastula [18] implants into the uterine wall of the mother, and is immediately followed by the development of the various organ systems (organogenesis [19]). This coordinated movement of cells results in a spatially organized embryo, and assembles the framework upon which future developmental processes will build the body. The term for an embryo undergoing gastrulation [7] is the gastrula [20], a term coined by Ernst Haeckel [21] Germany in 1872, and expanded upon in his 1874 Studien zur Gastraea-theorie (Studies for the Gastrea Theory). -
Stem-Cell-Based Embryo Models for Fundamental Research and Translation
REVIEW ARTICLE https://doi.org/10.1038/s41563-020-00829-9 Stem-cell-based embryo models for fundamental research and translation Jianping Fu 1,2,3 ✉ , Aryeh Warmflash 4,5 ✉ and Matthias P. Lutolf 6,7 ✉ Despite its importance, understanding the early phases of human development has been limited by availability of human sam- ples. The recent emergence of stem-cell-derived embryo models, a new field aiming to use stem cells to construct in vitro models to recapitulate snapshots of the development of the mammalian conceptus, opens up exciting opportunities to promote fundamental understanding of human development and advance reproductive and regenerative medicine. This Review provides a summary of the current knowledge of early mammalian development, using mouse and human conceptuses as models, and emphasizes their similarities and critical differences. We then highlight existing embryo models that mimic different aspects of mouse and human development. We further discuss bioengineering tools used for controlling multicellular interactions and self-organization critical for the development of these models. We conclude with a discussion of the important next steps and exciting future opportunities of stem-cell-derived embryo models for fundamental discovery and translation. he development of a multicellular organism from a single notable progress in studying non-human primate (NHP) monkey fertilized egg is a brilliant triumph of evolution that has fas- embryos10–12, whose developments are similar to humans. However, Tcinated generations of scientists (Box 1). Understanding our NHP monkey models remain costly, are difficult to modify geneti- own development is of particular fundamental and practical inter- cally, and have their own ethical challenges. -
Expression of the Cytokeratin Endo a Gene During Early Mouse
Proc. Natl. Acad. Sci. USA Vol. 82, pp. 8535-8539, December 1985 Developmental Biology Expression of the cytokeratin endo A gene during early mouse embryogenesis (nuclease S1 mapping/in situ hybridization) PHILIPPE DUPREY*, DOMINIQUE MORELLOt, MARC VASSEUR*, CHARLES BABINETt, HUBERT CONDAMINE*, PHILIPPE BROLET*, AND FRAN(OIS JACOB* *Unitd de Gdndtique Cellulaire du Col1ge de France et de 1'Institut Pasteur and tUnitd de Gdndtique des Mammifres, Institut Pasteur, 25, rue du Dr. Roux, 75724 Paris Cedex 15, France Contributed by Franqois Jacob, July 25, 1985 ABSTRACT Expression of cytokeratin endo A has been embryo analysis, matings between (i) female F1(C57BL/6 x analyzed during mouse blastocyst formation and embryonal CBA/J) and male F1(C57BL/6 x CBA/J) or (ii) female carcinoma cell differentiation. To study the regulation of endo BALB/c and male DBA/2 were used. A expression, nuclease S1 mapping experiments have been Embryos. Embryos (two-cell-stage, eight-cell-stage, or performed on RNA extracted from two-cell to 7.5-day embry- blastocysts) were recovered from virgin superovulated 3- to os. Low levels of endo A mRNA begin to be detectable in 5-week-old female mice that had been mated 3 or 4 days eight-cell embryos. The amount of this mRNA increases at the before. Embryos were washed extensively in a phosphate blastocyst stage, suggesting that endo A expression is regulated buffer medium and kept at -70'C before RNA extraction. at the mRNA level during blastocyst formation. At this stage, Cells. F9 and PCC3 are EC cell lines (18, 19) that were in situ hybridization studies show that endo A mRNA is present cultured in an undifferentiated state under standard condi- in the trophectoderm but not in the inner cell mass. -
Molecular Recording of Mammalian Embryogenesis Michelle M
ARTICLE https://doi.org/10.1038/s41586-019-1184-5 Molecular recording of mammalian embryogenesis Michelle M. Chan1,2,14, Zachary D. Smith3,4,5,14, Stefanie Grosswendt6, Helene Kretzmer6, Thomas M. Norman1,2, Britt Adamson1,2,13, Marco Jost1,2,7, Jeffrey J. Quinn1,2, Dian Yang1,2, Matthew G. Jones1,2,8, Alex Khodaverdian9,10, Nir Yosef9,10,11,12, Alexander Meissner3,4,6* & Jonathan S. Weissman1,2* Ontogeny describes the emergence of complex multicellular organisms from single totipotent cells. This field is particularly challenging in mammals, owing to the indeterminate relationship between self-renewal and differentiation, variation in progenitor field sizes, and internal gestation in these animals. Here we present a flexible, high-information, multi-channel molecular recorder with a single-cell readout and apply it as an evolving lineage tracer to assemble mouse cell-fate maps from fertilization through gastrulation. By combining lineage information with single-cell RNA sequencing profiles, we recapitulate canonical developmental relationships between different tissue types and reveal the nearly complete transcriptional convergence of endodermal cells of extra-embryonic and embryonic origins. Finally, we apply our cell-fate maps to estimate the number of embryonic progenitor cells and their degree of asymmetric partitioning during specification. Our approach enables massively parallel, high-resolution recording of lineage and other information in mammalian systems, which will facilitate the construction of a quantitative framework for understanding developmental processes. The development of a multicellular organism from a single cell is an aston- Here we generate and validate a method for simultaneously reporting ishing process. Classic lineage-tracing experiments using Caenorhabditis cellular state and lineage history in mice. -
Tet Proteins Influence the Balance Between PNAS PLUS Neuroectodermal and Mesodermal Fate Choice by Inhibiting Wnt Signaling
Tet proteins influence the balance between PNAS PLUS neuroectodermal and mesodermal fate choice by inhibiting Wnt signaling Xiang Lia,b, Xiaojing Yuea, William A. Pastora,1, Lizhu Linc, Romain Georgesa, Lukas Chaveza,2, Sylvia M. Evansc,d,3, and Anjana Raoa,b,e,f,3 aDivision of Signaling and Gene Expression, La Jolla Institute for Allergy and Immunology, La Jolla, CA 92037; bSanford Consortium for Regenerative Medicine, La Jolla, CA 92037; cSkaggs School of Pharmacy and Pharmaceutical Sciences, University of California at San Diego, La Jolla, CA 92093; dDepartment of Medicine, University of California at San Diego, La Jolla, CA 92093; eDepartment of Pharmacology, University of California at San Diego, La Jolla, CA 92093; and fMoores Cancer Center, University of California at San Diego, La Jolla, CA 92093 Contributed by Anjana Rao, November 2, 2016 (sent for review October 14, 2016; reviewed by Kristin K. Baldwin and Scott J. Bultman) TET-family dioxygenases catalyze conversion of 5-methylcytosine birth (17). Embryos lacking Tet1 and Tet3 only survive to embry- (5mC) to 5-hydroxymethylcytosine (5hmC) and oxidized methyl- onic day 10.5 (E10.5) and display poor forebrain formation and cytosines in DNA. Here, we show that mouse embryonic stem cells abnormal facial structures (18). The 5hmC generated by Tet family (mESCs), either lacking Tet3 alone or with triple deficiency of Tet1/2/3, dioxygenases is abundant in neurons (19) and may be critical for displayed impaired adoption of neural cell fate and concomitantly neural development (20). In Xenopus, Tet3 plays a vital function skewed toward cardiac mesodermal fate. Conversely, ectopic expres- in early eye and neural development by directly targeting several sion of Tet3 enhanced neural differentiation and limited cardiac key developmental genes (21). -
Molecular Recording of Mammalian Embryogenesis
HHS Public Access Author manuscript Author ManuscriptAuthor Manuscript Author Nature. Manuscript Author Author manuscript; Manuscript Author available in PMC 2020 May 16. Published in final edited form as: Nature. 2019 June ; 570(7759): 77–82. doi:10.1038/s41586-019-1184-5. Molecular recording of mammalian embryogenesis Michelle M. Chan1,2,*, Zachary D. Smith4,5,6,*, Stefanie Grosswendt7, Helene Kretzmer7, Thomas Norman1,2, Britt Adamson1,2, Marco Jost1,2,3, Jeffrey J. Quinn1,2, Dian Yang1,2, Matthew G. Jones1,2,8, Alex Khodaverdian9,10, Nir Yosef9,10,11,12, Alexander Meissner4,5,7, Jonathan S. Weissman1,2 1Department of Cellular and Molecular Pharmacology, University of California, San Francisco, San Francisco, CA, USA 2Howard Hughes Medical Institute, University of California, San Francisco, San Francisco, CA, USA 3Department of Microbiology and Immunology, University of California, San Francisco, San Francisco, CA, USA 4Broad Institute of MIT and Harvard, Cambridge, Massachusetts, USA 5Department of Stem Cell and Regenerative Biology, Harvard University, Cambridge, Massachusetts, USA 6Department of Molecular and Cellular Biology, Harvard University, Cambridge, Massachusetts, USA 7Department of Genome Regulation, Max Planck Institute for Molecular Genetics, Berlin 14195, Germany 8Integrative Program in Quantitative Biology, University of California, San Francisco, San Francisco, California, USA 9Department of Electrical Engineering and Computer Science, University of California, Berkeley, Berkeley, California, USA 10Center for Computational Biology, Berkeley, California, USA Users may view, print, copy, and download text and data-mine the content in such documents, for the purposes of academic research, subject always to the full Conditions of use:http://www.nature.com/authors/editorial_policies/license.html#terms Correspondence: [email protected] (J.S.W.), [email protected] (A.M.). -
Autonomy in the Development of Stem Cell-Derived Embryoids: Sprouting Blastocyst-Like Cysts, and Ethical Implications
cells Review Autonomy in the Development of Stem Cell-Derived Embryoids: Sprouting Blastocyst-Like Cysts, and Ethical Implications Hans-Werner Denker Universitätsklinikum, Institut für Anatomie, University Duisburg-Essen, Hufelandstr. 55, 45147 Essen, Germany; [email protected] Abstract: The experimental production of complex structures resembling mammalian embryos (e.g., blastoids, gastruloids) from pluripotent stem cells in vitro has become a booming research field. Since some of these embryoid models appear to reach a degree of complexity that may come close to viability, a broad discussion has set in with the aim to arrive at a consensus on the ethical implications with regard to acceptability of the use of this technology with human cells. The present text focuses on aspects of the gain of organismic wholeness of such stem cell-derived constructs, and of autonomy of self-organization, raised by recent reports on blastocyst-like cysts spontaneously budding in mouse stem cell cultures, and by previous reports on likewise spontaneous formation of gastrulating embryonic disc-like structures in primate models. Mechanisms of pattern (axis) formation in early embryogenesis are discussed in the context of self-organization of stem cell clusters. It is concluded that ethical aspects of development of organismic wholeness in the formation of embryoids need to receive more attention in the present discussions about new legal regulations in this field. Keywords: stem cells; embryoids; blastoids; gastruloids; blastocyst; expanded potential stem cells; development; morphogenesis; self-organization; ethics Citation: Denker, H.-W. Autonomy in the Development of Stem Cell-Derived Embryoids: Sprouting Blastocyst-Like Cysts, and Ethical 1. Introduction Implications.