ABSTRACT Investigation of Hadronic Resonances with STAR Sevil
Total Page:16
File Type:pdf, Size:1020Kb
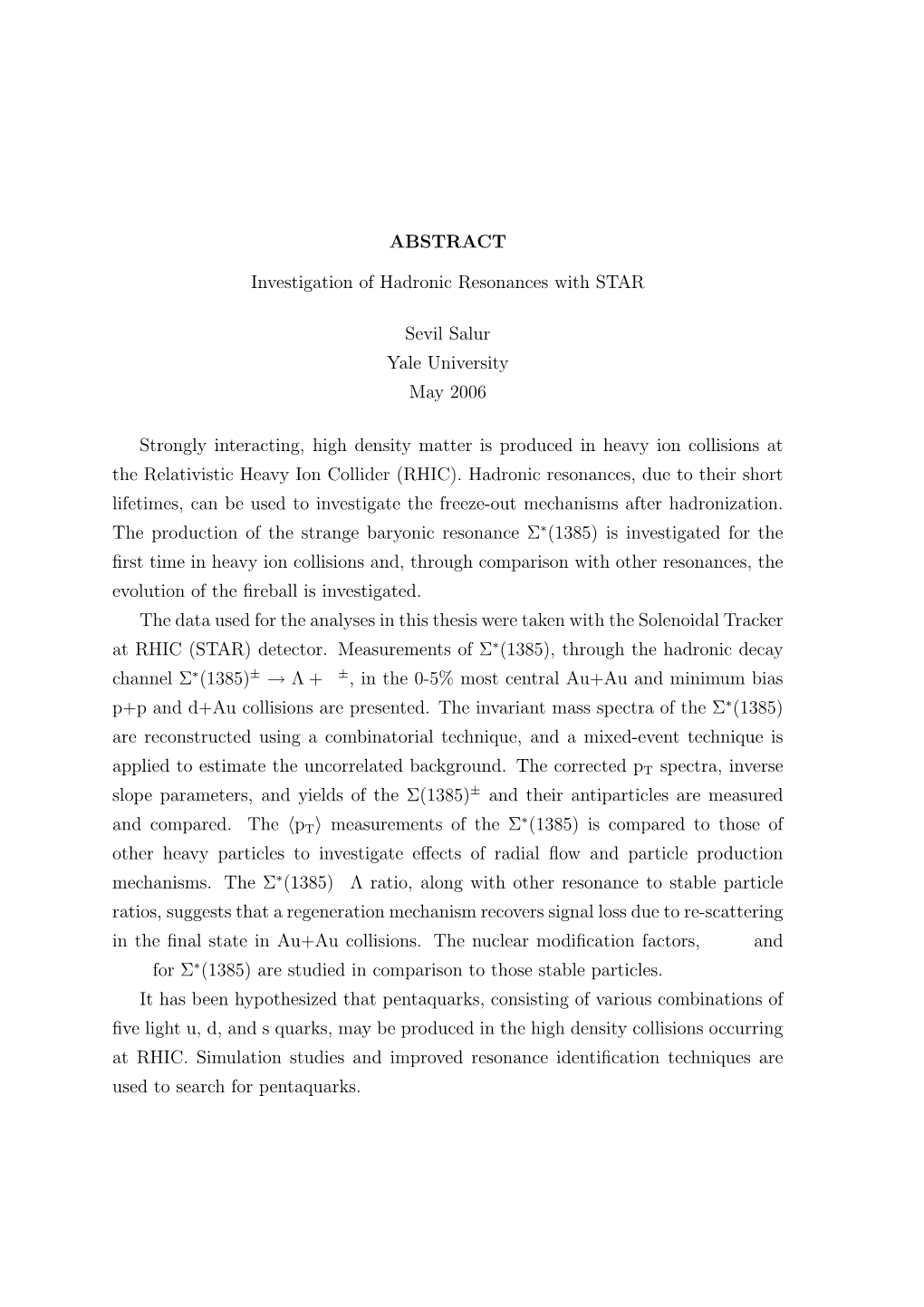
Load more
Recommended publications
-
J. Stroth Asked the Question: "Which Are the Experimental Evidences for a Long Mean Free Path of Phi Mesons in Medium?"
J. Stroth asked the question: "Which are the experimental evidences for a long mean free path of phi mesons in medium?" Answer by H. Stroebele ~~~~~~~~~~~~~~~~~~~ (based on the study of several publications on phi production and information provided by the theory friends of H. Stöcker ) Before trying to find an answer to this question, we need to specify what is meant with "long". The in medium cross section is equivalent to the mean free path. Thus we need to find out whether the (in medium) cross section is large (with respect to what?). The reference would be the cross sections of other mesons like pions or more specifically the omega meson. There is a further reference, namely the suppression of phi production and decay described in the OZI rule. A “blind” application of the OZI rule would give a cross section of the phi three orders of magnitude lower than that of the omega meson and correspondingly a "long" free mean path. In the following we shall look at the phi production cross sections in photon+p, pion+p, and p+p interactions. The total photoproduction cross sections of phi and omega mesons were measured in a bubble chamber experiment (J. Ballam et al., Phys. Rev. D 7, 3150, 1973), in which a cross section ratio of R(omega/phi) = 10 was found in the few GeV beam energy region. There are results on omega and phi production in p+p interactions available from SPESIII (Near- Threshold Production of omega mesons in the Reaction p p → p p omega", Phys.Rev.Lett. -
Understanding the J/Psi Production Mechanism at PHENIX Todd Kempel Iowa State University
Iowa State University Capstones, Theses and Graduate Theses and Dissertations Dissertations 2010 Understanding the J/psi Production Mechanism at PHENIX Todd Kempel Iowa State University Follow this and additional works at: https://lib.dr.iastate.edu/etd Part of the Physics Commons Recommended Citation Kempel, Todd, "Understanding the J/psi Production Mechanism at PHENIX" (2010). Graduate Theses and Dissertations. 11649. https://lib.dr.iastate.edu/etd/11649 This Dissertation is brought to you for free and open access by the Iowa State University Capstones, Theses and Dissertations at Iowa State University Digital Repository. It has been accepted for inclusion in Graduate Theses and Dissertations by an authorized administrator of Iowa State University Digital Repository. For more information, please contact [email protected]. Understanding the J= Production Mechanism at PHENIX by Todd Kempel A dissertation submitted to the graduate faculty in partial fulfillment of the requirements for the degree of DOCTOR OF PHILOSOPHY Major: Nuclear Physics Program of Study Committee: John G. Lajoie, Major Professor Kevin L De Laplante S¨orenA. Prell J¨orgSchmalian Kirill Tuchin Iowa State University Ames, Iowa 2010 Copyright c Todd Kempel, 2010. All rights reserved. ii TABLE OF CONTENTS LIST OF TABLES . v LIST OF FIGURES . vii CHAPTER 1. Overview . 1 CHAPTER 2. Quantum Chromodynamics . 3 2.1 The Standard Model . 3 2.2 Quarks and Gluons . 5 2.3 Asymptotic Freedom and Confinement . 6 CHAPTER 3. The Proton . 8 3.1 Cross-Sections and Luminosities . 8 3.2 Deep-Inelastic Scattering . 10 3.3 Structure Functions and Bjorken Scaling . 12 3.4 Altarelli-Parisi Evolution . -
On the Scent of Glue
On the scent of glue by Frank Close Although this work was not new Soon after the quark model was in has been intensifying over the last for the specialists, the underlying vented twenty years ago, people re several years. Where have all the message was clear. 'Because of its alized that it was in trouble. The Pauli flowers gone? inherent singularities, classical gen exclusion principle ruled out many eral relativity predicts its own down well known states, in particular the How colour forces work fall,' stated Hawking, 'just as the configuration of three identical classical picture of the atom was also strange quarks that formed the ome Electrical charges are the sources doomed'. ga-minus. The very hadron whose of electromagnetic forces. As every The meeting merited two closing discovery had confirmed the Eight schoolchild knows, opposite char lectures. For the cosmologists, Mar fold Way seemingly killed its off ges attract while like charges repel. tin Rees of Cambridge confessed to spring, the quark model. Quarks possess electric charge and finding the symposium an 'unusual In those days, many people were so feel electromagnetic forces. That experience'. For the particle physi reluctant to accept the idea of frac is why even electrically uncharged cists, John Ellis described particle tionally charged quarks which had particles like neutrons have electro physics and cosmology as having 'a never been seen. The Pauli paradox magnetic interactions; they contain brilliant past in front of them' — refer suggested that quarks were at best electrically charged constituents. ring to the new common interest in no more than a bookkeeping device, Quarks also have colour and it ap the primaeval Big Bang and its imme not physical particles. -
PARTICLE DECAYS the First Kaons from the New DAFNE Phi-Meson
PARTICLE DECAYS K for KLi The first kaons from the new DAFNE phi-meson factory at Frascati underline a fascinating chapter in the evolution of particle physics. As reported in the June issue (p7), in mid-April the new DAFNE phi-meson factory at Frascati began operation, with the KLOE detector looking at the physics. The DAFNE electron-positron collider operates at a total collision energy of 1020 MeV, the mass of the phi- meson, which prefers to decay into pairs of kaons.These decays provide a new stage to investigate CP violation, the subtle asymmetry that distinguishes between mat ter and antimatter. More knowledge of CP violation is the key to an increased understanding of both elemen tary particles and Big Bang cosmology. Since the discovery of CP violation in 1964, neutral kaons have been the classic scenario for CP violation, produced as secondary beams from accelerators. This is now changing as new CP violation scenarios open up with B particles, containing the fifth quark - "beauty", "bottom" or simply "b" (June p22). Although still on the neutral kaon beat, DAFNE offers attractive new experimental possibilities. Kaons pro duced via electron-positron annihilation are pure and uncontaminated by background, and having two kaons produced coherently opens up a new sector of preci sion kaon interferometry.The data are eagerly awaited. Strange decay At first sight the fact that the phi prefers to decay into pairs of kaons seems strange. The phi (1020 MeV) is only slightly heavier than a pair of neutral kaons (498 MeV each), and kinematically this decay is very constrained. -
Energy and System Size Dependence of Phi Meson Production in Cu+Cu and Au+Au Collisions
Lawrence Berkeley National Laboratory Lawrence Berkeley National Laboratory Title Energy and system size dependence of phi meson production in Cu+Cu and Au+Au collisions Permalink https://escholarship.org/uc/item/95k857j6 Authors Wissink, S.W. STAR Collaboration Publication Date 2009-08-26 eScholarship.org Powered by the California Digital Library University of California Energy and system size dependence of φ meson production in Cu+Cu and Au+Au collisions B. I. Abelev,1 M. M. Aggarwal,2 Z. Ahammed,3 B. D. Anderson,4 D. Arkhipkin,5 G. S. Averichev,6 Y. Bai,7 J. Balewski,8 O. Barannikova,1 L. S. Barnby,9 J. Baudot,10 S. Baumgart,11 D. R. Beavis,12 R. Bellwied,13 F. Benedosso,7 R. R. Betts,1 S. Bhardwaj,14 A. Bhasin,15 A. K. Bhati,2 H. Bichsel,16 J. Bielcik,17 J. Bielcikova,17 B. Biritz,18 L. C. Bland,12 M. Bombara,9 B. E. Bonner,19 M. Botje,7 J. Bouchet,4 E. Braidot,7 A. V. Brandin,20 S. Bueltmann,12 T. P. Burton,9 M. Bystersky,17 X. Z. Cai,21 H. Caines,11 M. Calder´on de la Barca S´anchez,22 J. Callner,1 O. Catu,11 D. Cebra,22 R. Cendejas,18 M. C. Cervantes,23 Z. Chajecki,24 P. Chaloupka,17 S. Chattopadhyay,3 H. F. Chen,25 J. H. Chen,21 J. Y. Chen,26 J. Cheng,27 M. Cherney,28 A. Chikanian,11 K. E. Choi,29 W. Christie,12 S. U. Chung,12 R. F. Clarke,23 M. J. -
Phi Meson in Dense Matter
PHYSICAL REVIEW C VOLUME 45, NUMBER 3 MARCH 1992 Phi meson in dense matter * C. M. Ko, P. Levai, and X. J. Qiu Cyclotron Institute and Physics Department, Texas A &M University, College Station, Texas 77843 C. T. Li Physics Department, National Taiwan University, Taipei, Taiwan 10764, China {Received 3 September 1991) The effect of the kaon loop correction to the property of a phi meson in dense matter is studied in the vector dominance model. Using the density-dependent kaon effective mass determined from the linear chiral perturbation theory, we find that with increasing baryon density the phi meson mass is reduced slightly while its width is broadened drastically. PACS number(s): 21.30.+y, 21.90.+f In relativistic heavy-ion collisions, the nuclear matter density-dependent kaon effective mass as given by the can be compressed to densities which are many times that linear chiral perturbation theory [5,6]. in normal nuclei. This has recently generated great in- According to Refs. [5,6], the kaon effective mass in the terests in theoretical studies of hadron properties under medium can be expressed as extreme conditions [1]. In studies with both ' 1/2 Nambu —Jona-Lasinio model and sum rules pa [2] QCD m& =m& 1— [1,3], it has been found that hadron masses in general de- Pc crease with increasing density as a result of the partial restoration of chiral symmetry. The masses of pseudo- with scalar Goldstone bosons such as pion and kaon, however, 2 2 p m )yKN (2) do not depend much on the density [2,4]. -
Proposal to Jefferson Lab PAC39 Exclusive Phi Meson
Proposal to Jefferson Lab PAC39 Exclusive Phi Meson Electroproduction with CLAS12 H. Avakian,1 J. Ball,2 A. Biselli,3 V. Burkert,1 R. Dupr,2 L. Elouadrhiri,1 1 1, 4 5, 6 5, R. Ent, F.{X. Girod, ∗ S. Goloskokov, B. Guegan, M. Guidal, ∗ 5 7 8 5 2 6, H.{S. Jo, K. Joo, P. Kroll, A Marti, H. Moutarde, A. Kubarovsky, ∗ 1, 5 5 1 5 V. Kubarovsky, ∗ C. Munoz Camacho, S. Niccolai, K. Park, R. Paremuzyan, 2 2 6, 5 5 1 6, S. Procureur, F. Sabati´e, N. Saylor, D. Sokhan, S. Stepanyan, P. Stoler, y 7 9 1, 1 M. Ungaro, E. Voutier, C. Weiss, y D. Weygand, and the CLAS Collaboration 1Jefferson Lab, Newport News, VA 23606, USA 2IRFU/SPhN, Saclay, France 3Fairfield University 4Joint Institute for Nuclear Research, Dubna, Russia 5Institut de Physique Nucleaire Orsay, France 6Rensselaer Polytechnic Institute 7Department of Physics, University of Connecticut, Storrs, CT 06269, USA 8Wuppertal University, Wuppertal, Germany 9LPSC Grenoble, France ∗Spokespersons ySpokespersons,Contact persons 2 Summary We propose a measurement of exclusive φ meson electroproduction on the proton, ep ! e0 + φ + p, at 11 GeV beam energy with the CLAS12 detector. The kinematic range extends 2 2 2 in W from 2{5 GeV, Q from 1{12 GeV , and jt − tminj from near zero to ∼ 4 GeV , the precise limits depending on the specific values of the other variables. The φ will be detected + through the K K− and (for the first time) the KSKL mode, which allows for an independent test of the cross section extraction. -
Measurements of Phi Meson Production in Relativistic Heavy- Ion Collisions at the BNL Relativistic Heavy Ion Collider (RHIC)
Measurements of phi meson production in relativistic heavy- ion collisions at the BNL Relativistic Heavy Ion Collider (RHIC) The MIT Faculty has made this article openly available. Please share how this access benefits you. Your story matters. Citation STAR Collaboration et al. “Measurements of phi meson production in relativistic heavy-ion collisions at the BNL Relativistic Heavy Ion Collider (RHIC).” Physical Review C 79.6 (2009): 064903. © 2009 The American Physical Society As Published http://dx.doi.org/10.1103/PhysRevC.79.064903 Publisher American Physical Society Version Final published version Citable link http://hdl.handle.net/1721.1/51838 Terms of Use Article is made available in accordance with the publisher's policy and may be subject to US copyright law. Please refer to the publisher's site for terms of use. PHYSICAL REVIEW C 79, 064903 (2009) Measurements of φ meson production in relativistic heavy-ion collisions at the BNL Relativistic Heavy Ion Collider (RHIC) B. I. Abelev,9 M. M. Aggarwal,30 Z. Ahammed,46 B. D. Anderson,19 D. Arkhipkin,13 G. S. Averichev,12 Y. Bai, 28 J. Balewski,23 O. Barannikova,9 L. S. Barnby,2 J. Baudot,17 S. Baumgart,51 D. R. Beavis,3 R. Bellwied,49 F. Benedosso,28 R. R. Betts,9 S. Bhardwaj,35 A. Bhasin,18 A. K. Bhati,30 H. Bichsel,48 J. Bielcik,11 J. Bielcikova,11 B. Biritz,6 L. C. Bland,3 S.-L. Blyth,22 M. Bombara,2 B. E. Bonner,36 M. Botje,28 J. Bouchet,19 E. Braidot,28 A. V. -
Phi Meson Production in Heavy-Ion Collisions at SIS Energiesprovided by CERN Document Server
View metadata, citation and similar papers at core.ac.uk brought to you by CORE Phi Meson Production in Heavy-Ion Collisions at SIS Energiesprovided by CERN Document Server W. S. Chung1,G.Q.Li1,2, and C. M. Ko1 1Department of Physics and Cyclotron Institute, Texas A&M University, College Station, Texas 77843, U.S.A. 2Department of Physics, State University of New York at Stony Brook, Stony Brook, New York 11794, U.S.A. Abstract Phi meson production in heavy-ion collisions at SIS/GSI energies (∼ 2 GeV/nucleon) is studied in the relativistic transport model. We include contributions from baryon-baryon, pion-baryon, and kaon-antikaon collisions. The cross sections for the first two processes are obtained in an one-boson- exchange model, while that for the last process is taken to be of Breit-Wigner form through the phi meson resonance. The dominant contribution to phi meson production in heavy ion collisions at these energies is found to come from secondary pion-nucleon collisions. Effects due to medium modifications of kaon masses are also studied and are found to reduce the phi meson yield by about a factor of two, mainly because of increased phi decay width as a result of dropping kaon-antikaon masses. In this case, the φ/K− ratio is about 4%, which is a factor of 2-3 below preliminary experimental data from the FOPI collaboration at GSI. Including also the reduction of phi meson mass in medium increases this ratio to about 8%, which is then in reasonable agreement with the data. -
Coherent Ρ Meson Electroproduction Off Deuteron
FLORIDA INTERNATIONAL UNIVERSITY Miami, Florida COHERENT RHO MESON ELECTROPRODUCTION OFF DEUTERON A dissertation submitted in partial fulfillment of the requirements for the degree of DOCTOR OF PHILOSOPHY in PHYSICS by Atilla Gönenç 2009 1 To: Dean Kenneth Furton College of Arts and Sciences This dissertation, written by Atilla Gönenç, and entitled Coherent Rho Meson Electroproduction off Deuteron, having been approved in respect to style and intellectual content, is referred to you for judgment. We have read this dissertation and recommend that it be approved. _______________________________________ Werner U. Boeglin _______________________________________ Cem Karayalcin _______________________________________ Laird Kramer _______________________________________ Misak Sargsian _______________________________________ Brian A. Raue, Major Professor Date of Defense: June 24, 2008 The dissertation of Atilla Gönenç is approved. _______________________________________ Dean Kenneth Furton College of Arts and Sciences _______________________________________ Dean George Walker University Graduate School Florida International University, 2009 ii © Copyright 2009 by Atilla Gönenç All rights reserved. iii DEDICATION This dissertation is dedicated to my wonderful parents, Tulin and Hasim, for their love and endless support… iv ACKNOWLEDGMENTS Though only my name appears on the cover of this dissertation, a great many people have contributed to its creation. The writing of this dissertation has been truly a challenge and I owe my thanks to all those who gave me the possibility to complete it. My deepest gratitude is to my advisor, Dr. Brian Raue. His guidance, patience and support helped me overcome many crisis situations and finish this dissertation. Most importantly, none of this would have been possible without the love and patients of my family. My immediate family, to whom this dissertation is dedicated to, has been source of love, support and strength all these years. -
Hyperon Puzzle and RMF Models with Scaled Hadron Masses and Coupling Constants
Hyperon puzzle and RMF models with scaled hadron masses and coupling constants Evgeni E. Kolomeitsev (University of Matej Bel, Slovakia) K.A. Maslov and D.N. Voskresensky (MEPhI, Moscow) “Hyperon puzzle” and constraints on the nuclear EoS maximum mass of a neutron star constraint on pressure from HIC constraint from direct Urca (DU) processes “hyperon puzzle” Cut mechanism for hardening the nucleon EoS. Non-linear Walecka model: Play with a scalar-field potential Scaling of meson masses and coupling constants Solution of the hyperons puzzle in neutron stars D baryons Neutron star mass charts 1993 [Thorsett et al, ApJ 405] 2012 2000 http://www.stellarcollapse.org/ Constraint on the stiffness of nuclear EoS [Danielewicz, Lacey, Lynch, Science 298, 1592 (2002)] directed and elliptic flow of particles in heavy-ion collisions range for the pressure of isospin symmetrical matter at T=0 maximum NS mass >1.98 Msol requires stiff EoS restrict the pressure from above! Neutron star cooling and direct Urca reactions DU: MU: DU process schould be „exotics“ (if DU starts it is dificult to stop it) weak constraint strong constraint [Blaschke, Grigorian, Voskresensky A&A 424 (2004) 979] constraint on the symmetry energy [EEK, Voskresensky NPA759 (2005) 373] “Hyperon puzzle” If we allow for a population of new Fermi seas (hyperon, D baryons, …) EoS will be softer and the NS will be smaller [Rijken, Schulze, EPJA52 (2016) 21] [Dapo, Schaefer, Wambach PRC 81 (2010) 035803] Simple solutions: -- make nuclear EoS as stiff as possible [flow constraint] -- suppress hyperon population (increase repulsion/reduce attraction) against phenomenology of YN,NN,YY interaction in vacuum +hypernuclear physics various RMF models nucleonic stars hyperonic stars [Weissenborn et al., NPA 881 (2012) 62] “Cut” mechanism for hardening the nuclear EoS. -
Phi Meson Production in Relativistic Heavy Ion Collisions
Phi Meson Production in Relativistic Heavy Ion Collisions S. Pal, C. M. Ko, and Z. W. Lin One possible signal for the quark-gluon plasma formed in relativistic heavy ion collisions is the enhanced production of strange particles such as the phi meson [1]. Since phi meson is not a stable particle, it can only be detected via its decay product of KK+− or µµ+−. The rescattering of K + and K − in the hadronic matter, however, prevents the reconstruction of all phi mesons produced in heavy ion collisions. This makes the µµ+−, which does not suffer final-state interactions, a more reliable way to extract the phi meson yield. Recent experiments at the CERN/SPS have, indeed, shown that the number of phi meson reconstructed from the µµ+− channel [2] Figure 1: Rapidity distribution of phi meson reconstructed exceeds by a factor between two and four from from K+K- (solid curves) and Φ+Φ- channel (dashed curves) +− for Pb+Pb collisions at 158A GeV at an impact parameter that reconstructed from the KK channel [3]. of b#3.5 fm in the AMPT model. The results are for To understand these experimental results, we without (thin curves) and with (thick curves) in-medium mass modifications. The dotted line corresponds to phi have extended a multiphase transport (AMPT) mesons from the dimuon channel with in-medium masses model [4], that takes into account both initial and with the phi meson number from initial string partonic and final hadronic interactions, to fragmentation increased by a factor of two. The solid circles are the NA49 experimental data [3] from the K+ K- include phi meson production, scattering, and channel.