D Meson Reconstruction at Star Using the Silicon Vertex Tracker (Svt) Sarah Lapointe Wayne State University
Total Page:16
File Type:pdf, Size:1020Kb
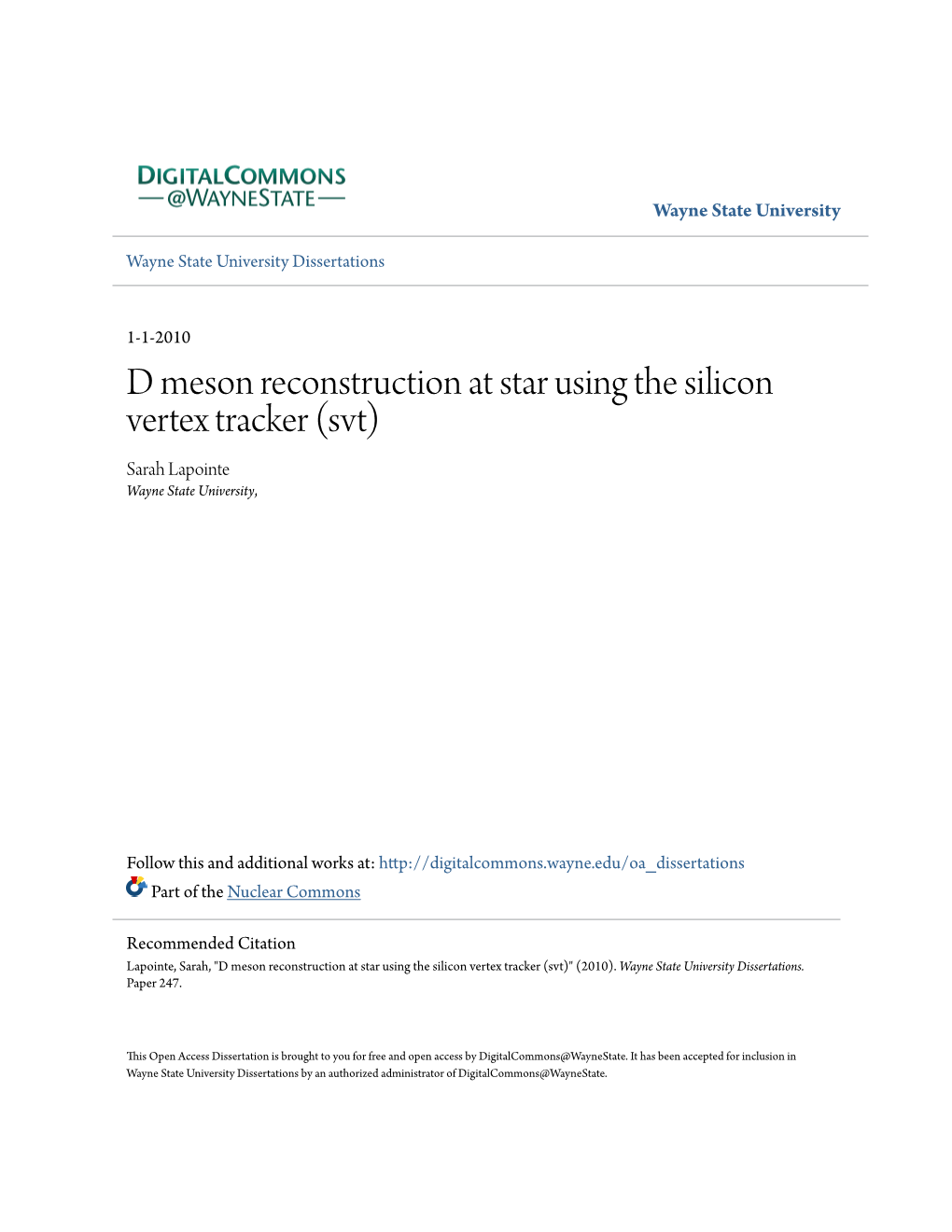
Load more
Recommended publications
-
Spectator Model in D Meson Decays
Transaction B: Mechanical Engineering Vol. 16, No. 2, pp. 140{148 c Sharif University of Technology, April 2009 Spectator Model in D Meson Decays H. Mehrban1 Abstract. In this research, the e ective Hamiltonian theory is described and applied to the calculation of current-current (Q1;2) and QCD penguin (Q3; ;6) decay rates. The channels of charm quark decay in the quark levels are: c ! dud, c ! dus, c ! sud and c ! sus where the channel c ! sud is dominant. The total decay rates of the hadronic of charm quark in the e ective Hamiltonian theory are calculated. The decay rates of D meson decays according to Spectator Quark Model (SQM) are investigated for the calculation of D meson decays. It is intended to make the transition from decay rates at the quark level to D meson decay rates for two body hadronic decays, D ! h1h2. By means of that, the modes of nonleptonic D ! PV , D ! PP , D ! VV decays where V and P are light vector with J P = 0 and pseudoscalar with J P = 1 mesons are analyzed, respectively. So, the total decay rates of the hadronic of charm quark in the e ective Hamiltonian theory, according to Colour Favoured (C-F) and Colour Suppressed (C-S) are obtained. Then the amplitude of the Colour Favoured and Colour Suppressed (F-S) processes are added and their decay rates are obtained. Using the spectator model, the branching ratio of some D meson decays are derived as well. Keywords: E ective Hamilton; c quark; D meson; Spectator model; Hadronic; Colour favoured; Colour suppressed. -
HADRONIC DECAYS of the Ds MESON and a MODEL-INDEPENDENT DETERMINATION of the BRANCHING FRACTION
SLAC-R-95-470 UC-414 HADRONIC DECAYS OF THE Ds MESON AND A MODEL-INDEPENDENT DETERMINATION OF THE BRANCHING FRACTION FOR THE Ds DECAY OF THE PHI PI* John Nicholas Synodinos Stanford Linear Accelerator Center Stanford University Stanford, California 94309 To the memory of my parents, July 1995 Alexander and Cnryssoula Synodinos Prepared for the Department of Energy under contract number DE-AC03-76SF00515 Printed in the United States of America. Available from the National Technical Information Service, U.S. Department of Commerce, 5285 Port Royal Road, Springfield, Virginia 22161. *Ph.D. thesis 0lSr^BUTlONoFT^ J "OFTH/3DOCUM*.~ ^ Abstract Acknowledgements During the running periods of the years 1992, 1993, 1994 the BES experiment at This work would not have been possible without the continuing guidance and support the Beijing Electron Positron Collider (BEPC) collected 22.9 ± 0.7pt_1 of data at an from BES collaborators, fellow graduate students, family members and friends. It is energy of 4.03 GeV, which corresponds to a local peak for e+e~ —* DfD~ production. difficult to give proper recognition to all of them, and I wish to apologize up front to Four Ds hadronic decay modes were tagged: anyone whose contributions I have overlooked in these acknowledgements. I owe many thanks to my advisor, Jonathan Dorfan, for providing me with guid• • D -> <t>w; <t> -* K+K~ s ance and encouragement. It was a priviledge to have been his graduate student. I wish to thank Bill Dunwoodie for his day to day advice. His understanding of physics • Ds~> 7F(892)°A'; 7F°(892) -> K~JT+ and his willingness to share his knowledge have been essential to the completion of • D -» WK; ~K° -> -K+TT- s this analysis. -
J. Stroth Asked the Question: "Which Are the Experimental Evidences for a Long Mean Free Path of Phi Mesons in Medium?"
J. Stroth asked the question: "Which are the experimental evidences for a long mean free path of phi mesons in medium?" Answer by H. Stroebele ~~~~~~~~~~~~~~~~~~~ (based on the study of several publications on phi production and information provided by the theory friends of H. Stöcker ) Before trying to find an answer to this question, we need to specify what is meant with "long". The in medium cross section is equivalent to the mean free path. Thus we need to find out whether the (in medium) cross section is large (with respect to what?). The reference would be the cross sections of other mesons like pions or more specifically the omega meson. There is a further reference, namely the suppression of phi production and decay described in the OZI rule. A “blind” application of the OZI rule would give a cross section of the phi three orders of magnitude lower than that of the omega meson and correspondingly a "long" free mean path. In the following we shall look at the phi production cross sections in photon+p, pion+p, and p+p interactions. The total photoproduction cross sections of phi and omega mesons were measured in a bubble chamber experiment (J. Ballam et al., Phys. Rev. D 7, 3150, 1973), in which a cross section ratio of R(omega/phi) = 10 was found in the few GeV beam energy region. There are results on omega and phi production in p+p interactions available from SPESIII (Near- Threshold Production of omega mesons in the Reaction p p → p p omega", Phys.Rev.Lett. -
Understanding the J/Psi Production Mechanism at PHENIX Todd Kempel Iowa State University
Iowa State University Capstones, Theses and Graduate Theses and Dissertations Dissertations 2010 Understanding the J/psi Production Mechanism at PHENIX Todd Kempel Iowa State University Follow this and additional works at: https://lib.dr.iastate.edu/etd Part of the Physics Commons Recommended Citation Kempel, Todd, "Understanding the J/psi Production Mechanism at PHENIX" (2010). Graduate Theses and Dissertations. 11649. https://lib.dr.iastate.edu/etd/11649 This Dissertation is brought to you for free and open access by the Iowa State University Capstones, Theses and Dissertations at Iowa State University Digital Repository. It has been accepted for inclusion in Graduate Theses and Dissertations by an authorized administrator of Iowa State University Digital Repository. For more information, please contact [email protected]. Understanding the J= Production Mechanism at PHENIX by Todd Kempel A dissertation submitted to the graduate faculty in partial fulfillment of the requirements for the degree of DOCTOR OF PHILOSOPHY Major: Nuclear Physics Program of Study Committee: John G. Lajoie, Major Professor Kevin L De Laplante S¨orenA. Prell J¨orgSchmalian Kirill Tuchin Iowa State University Ames, Iowa 2010 Copyright c Todd Kempel, 2010. All rights reserved. ii TABLE OF CONTENTS LIST OF TABLES . v LIST OF FIGURES . vii CHAPTER 1. Overview . 1 CHAPTER 2. Quantum Chromodynamics . 3 2.1 The Standard Model . 3 2.2 Quarks and Gluons . 5 2.3 Asymptotic Freedom and Confinement . 6 CHAPTER 3. The Proton . 8 3.1 Cross-Sections and Luminosities . 8 3.2 Deep-Inelastic Scattering . 10 3.3 Structure Functions and Bjorken Scaling . 12 3.4 Altarelli-Parisi Evolution . -
Effective Field Theory for Doubly Heavy Baryons and Lattice
E®ective Field Theory for Doubly Heavy Baryons and Lattice QCD by Jie Hu Department of Physics Duke University Date: Approved: Thomas C. Mehen, Supervisor Shailesh Chandrasekharan Albert M. Chang Haiyan Gao Mark C. Kruse Dissertation submitted in partial ful¯llment of the requirements for the degree of Doctor of Philosophy in the Department of Physics in the Graduate School of Duke University 2009 ABSTRACT E®ective Field Theory for Doubly Heavy Baryons and Lattice QCD by Jie Hu Department of Physics Duke University Date: Approved: Thomas C. Mehen, Supervisor Shailesh Chandrasekharan Albert M. Chang Haiyan Gao Mark C. Kruse An abstract of a dissertation submitted in partial ful¯llment of the requirements for the degree of Doctor of Philosophy in the Department of Physics in the Graduate School of Duke University 2009 Copyright °c 2009 by Jie Hu All rights reserved. Abstract In this thesis, we study e®ective ¯eld theories for doubly heavy baryons and lattice QCD. We construct a chiral Lagrangian for doubly heavy baryons and heavy mesons that is invariant under heavy quark-diquark symmetry at leading order and includes the leading O(1=mQ) symmetry violating operators. The theory is used to predict 3 the electromagnetic decay width of the J = 2 member of the ground state doubly heavy baryon doublet. Numerical estimates are provided for doubly charm baryons. We also calculate chiral corrections to doubly heavy baryon masses and strong de- cay widths of low lying excited doubly heavy baryons. We derive the couplings of heavy diquarks to weak currents in the limit of heavy quark-diquark symmetry, and construct the chiral Lagrangian for doubly heavy baryons coupled to weak currents. -
On the Scent of Glue
On the scent of glue by Frank Close Although this work was not new Soon after the quark model was in has been intensifying over the last for the specialists, the underlying vented twenty years ago, people re several years. Where have all the message was clear. 'Because of its alized that it was in trouble. The Pauli flowers gone? inherent singularities, classical gen exclusion principle ruled out many eral relativity predicts its own down well known states, in particular the How colour forces work fall,' stated Hawking, 'just as the configuration of three identical classical picture of the atom was also strange quarks that formed the ome Electrical charges are the sources doomed'. ga-minus. The very hadron whose of electromagnetic forces. As every The meeting merited two closing discovery had confirmed the Eight schoolchild knows, opposite char lectures. For the cosmologists, Mar fold Way seemingly killed its off ges attract while like charges repel. tin Rees of Cambridge confessed to spring, the quark model. Quarks possess electric charge and finding the symposium an 'unusual In those days, many people were so feel electromagnetic forces. That experience'. For the particle physi reluctant to accept the idea of frac is why even electrically uncharged cists, John Ellis described particle tionally charged quarks which had particles like neutrons have electro physics and cosmology as having 'a never been seen. The Pauli paradox magnetic interactions; they contain brilliant past in front of them' — refer suggested that quarks were at best electrically charged constituents. ring to the new common interest in no more than a bookkeeping device, Quarks also have colour and it ap the primaeval Big Bang and its imme not physical particles. -
Charm Meson Decays 3 for Charm Production Is Relatively High, Σ(D0D¯ 0) = 3.66 0.03 0.06 Nb and Σ(D+D−) = 2.91 0.03 0.05 Nb (6)
Charm Meson Decays 1 Charm Meson Decays Marina Artusoa, Brian Meadowsb, Alexey A Petrovc,d aSyracuse University, Syracuse, NY 13244, USA bUniversity of Cincinnati, Cincinnati, OH 45221, USA cWayne State University, Detroit, MI 48201, USA dMCTP, University of Michigan, Ann Arbor, MI 48109, USA Key Words charmed quark, charmed meson, weak decays, CP-violation Abstract We review some recent developments in charm meson physics. In particular, we discuss theoretical predictions and experimental measurements of charmed meson decays to lep- tonic, semileptonic, and hadronic final states and implications of such measurements to searches 0 for new physics. We discuss D0 − D -mixing and CP-violation in charm, and discuss future experimental prospects and theoretical challenges in this area. CONTENTS INTRODUCTION .................................... 2 LEPTONIC AND SEMILEPTONIC DECAYS .................... 3 Theoretical Predictions for the Decay Constant ...................... 5 Experimental Determinations of fD ............................. 5 Constraints on New Physics from fD ............................ 6 Absolute Branching Fractions for Semileptonic D Decays ................. 6 Form Factors For The Decays D → K(π)ℓν ....................... 7 The CKM Matrix ...................................... 9 Form Factors in Semileptonic D → V ℓν Decays ...................... 9 arXiv:0802.2934v1 [hep-ph] 20 Feb 2008 RARE AND RADIATIVE DECAYS .......................... 10 Theoretical Motivation .................................... 10 Experimental Information -
B Meson Decays Marina Artuso1, Elisabetta Barberio2 and Sheldon Stone*1
Review Open Access B meson decays Marina Artuso1, Elisabetta Barberio2 and Sheldon Stone*1 Address: 1Department of Physics, Syracuse University, Syracuse, NY 13244, USA and 2School of Physics, University of Melbourne, Victoria 3010, Australia Email: Marina Artuso - [email protected]; Elisabetta Barberio - [email protected]; Sheldon Stone* - [email protected] * Corresponding author Published: 20 February 2009 Received: 20 February 2009 Accepted: 20 February 2009 PMC Physics A 2009, 3:3 doi:10.1186/1754-0410-3-3 This article is available from: http://www.physmathcentral.com/1754-0410/3/3 © 2009 Stone et al This is an Open Access article distributed under the terms of the Creative Commons Attribution License (http://creativecommons.org/ licenses/by/2.0), which permits unrestricted use, distribution, and reproduction in any medium, provided the original work is properly cited. Abstract We discuss the most important Physics thus far extracted from studies of B meson decays. Measurements of the four CP violating angles accessible in B decay are reviewed as well as direct CP violation. A detailed discussion of the measurements of the CKM elements Vcb and Vub from semileptonic decays is given, and the differences between resulting values using inclusive decays versus exclusive decays is discussed. Measurements of "rare" decays are also reviewed. We point out where CP violating and rare decays could lead to observations of physics beyond that of the Standard Model in future experiments. If such physics is found by directly observation of new particles, e.g. in LHC experiments, B decays can play a decisive role in interpreting the nature of these particles. -
Masses of Scalar and Axial-Vector B Mesons Revisited
Eur. Phys. J. C (2017) 77:668 DOI 10.1140/epjc/s10052-017-5252-4 Regular Article - Theoretical Physics Masses of scalar and axial-vector B mesons revisited Hai-Yang Cheng1, Fu-Sheng Yu2,a 1 Institute of Physics, Academia Sinica, Taipei 115, Taiwan, Republic of China 2 School of Nuclear Science and Technology, Lanzhou University, Lanzhou 730000, People’s Republic of China Received: 2 August 2017 / Accepted: 22 September 2017 / Published online: 7 October 2017 © The Author(s) 2017. This article is an open access publication Abstract The SU(3) quark model encounters a great chal- 1 Introduction lenge in describing even-parity mesons. Specifically, the qq¯ quark model has difficulties in understanding the light Although the SU(3) quark model has been applied success- scalar mesons below 1 GeV, scalar and axial-vector charmed fully to describe the properties of hadrons such as pseu- mesons and 1+ charmonium-like state X(3872). A common doscalar and vector mesons, octet and decuplet baryons, it wisdom for the resolution of these difficulties lies on the often encounters a great challenge in understanding even- coupled channel effects which will distort the quark model parity mesons, especially scalar ones. Take vector mesons as calculations. In this work, we focus on the near mass degen- an example and consider the octet vector ones: ρ,ω, K ∗,φ. ∗ ∗0 eracy of scalar charmed mesons, Ds0 and D0 , and its impli- Since the constituent strange quark is heavier than the up or cations. Within the framework of heavy meson chiral pertur- down quark by 150 MeV, one will expect the mass hierarchy bation theory, we show that near degeneracy can be quali- pattern mφ > m K ∗ > mρ ∼ mω, which is borne out by tatively understood as a consequence of self-energy effects experiment. -
PARTICLE DECAYS the First Kaons from the New DAFNE Phi-Meson
PARTICLE DECAYS K for KLi The first kaons from the new DAFNE phi-meson factory at Frascati underline a fascinating chapter in the evolution of particle physics. As reported in the June issue (p7), in mid-April the new DAFNE phi-meson factory at Frascati began operation, with the KLOE detector looking at the physics. The DAFNE electron-positron collider operates at a total collision energy of 1020 MeV, the mass of the phi- meson, which prefers to decay into pairs of kaons.These decays provide a new stage to investigate CP violation, the subtle asymmetry that distinguishes between mat ter and antimatter. More knowledge of CP violation is the key to an increased understanding of both elemen tary particles and Big Bang cosmology. Since the discovery of CP violation in 1964, neutral kaons have been the classic scenario for CP violation, produced as secondary beams from accelerators. This is now changing as new CP violation scenarios open up with B particles, containing the fifth quark - "beauty", "bottom" or simply "b" (June p22). Although still on the neutral kaon beat, DAFNE offers attractive new experimental possibilities. Kaons pro duced via electron-positron annihilation are pure and uncontaminated by background, and having two kaons produced coherently opens up a new sector of preci sion kaon interferometry.The data are eagerly awaited. Strange decay At first sight the fact that the phi prefers to decay into pairs of kaons seems strange. The phi (1020 MeV) is only slightly heavier than a pair of neutral kaons (498 MeV each), and kinematically this decay is very constrained. -
D-Meson Production in P-Pb Collisions at √ Snn = 5.02 Tev and in Pp
PHYSICAL REVIEW C 94, 054908 (2016) √ √ D-meson production in p-Pb collisions at sNN = 5.02 TeV and in pp collisions at s = 7TeV J. Adam et al.∗ (ALICE Collaboration) (Received 7 June 2016; published 23 November 2016) Background: In the context of the investigation of the quark gluon plasma produced in heavy-ion collisions, hadrons containing heavy (charm or beauty) quarks play a special role for the characterization of the hot and dense medium created in the interaction. The measurement of the production of charm and beauty hadrons in proton– proton collisions, besides providing the necessary reference for the studies in heavy-ion reactions, constitutes an important test of perturbative quantum chromodynamics (pQCD) calculations. Heavy-flavor production in proton–nucleus collisions is sensitive to the various effects related to the presence of nuclei in the colliding system, commonly denoted cold-nuclear-matter effects. Most of these effects are expected to modify open-charm production at low transverse momenta (pT) and, so far, no measurement of D-meson production down to zero transverse momentum was available at mid-rapidity at the energies attained at the CERN Large Hadron Collider (LHC). Purpose: The measurements of the production cross sections of promptly produced charmed mesons in p-Pb collisions at the LHC down to pT = 0 and the comparison to the results from pp interactions are aimed at the assessment of cold-nuclear-matter effects on open-charm production, which is crucial for the interpretation of the results from Pb-Pb collisions. D0 D+ D∗+ D+ p Methods: The prompt charmed mesons √, , ,and s were measured at mid-rapidity in -Pb collisions at a center-of-mass energy per nucleon pair sNN = 5.02 TeV with the ALICE detector at the LHC. -
Energy and System Size Dependence of Phi Meson Production in Cu+Cu and Au+Au Collisions
Lawrence Berkeley National Laboratory Lawrence Berkeley National Laboratory Title Energy and system size dependence of phi meson production in Cu+Cu and Au+Au collisions Permalink https://escholarship.org/uc/item/95k857j6 Authors Wissink, S.W. STAR Collaboration Publication Date 2009-08-26 eScholarship.org Powered by the California Digital Library University of California Energy and system size dependence of φ meson production in Cu+Cu and Au+Au collisions B. I. Abelev,1 M. M. Aggarwal,2 Z. Ahammed,3 B. D. Anderson,4 D. Arkhipkin,5 G. S. Averichev,6 Y. Bai,7 J. Balewski,8 O. Barannikova,1 L. S. Barnby,9 J. Baudot,10 S. Baumgart,11 D. R. Beavis,12 R. Bellwied,13 F. Benedosso,7 R. R. Betts,1 S. Bhardwaj,14 A. Bhasin,15 A. K. Bhati,2 H. Bichsel,16 J. Bielcik,17 J. Bielcikova,17 B. Biritz,18 L. C. Bland,12 M. Bombara,9 B. E. Bonner,19 M. Botje,7 J. Bouchet,4 E. Braidot,7 A. V. Brandin,20 S. Bueltmann,12 T. P. Burton,9 M. Bystersky,17 X. Z. Cai,21 H. Caines,11 M. Calder´on de la Barca S´anchez,22 J. Callner,1 O. Catu,11 D. Cebra,22 R. Cendejas,18 M. C. Cervantes,23 Z. Chajecki,24 P. Chaloupka,17 S. Chattopadhyay,3 H. F. Chen,25 J. H. Chen,21 J. Y. Chen,26 J. Cheng,27 M. Cherney,28 A. Chikanian,11 K. E. Choi,29 W. Christie,12 S. U. Chung,12 R. F. Clarke,23 M. J.