DNA Sequence Variation in the Wingless Gene Product in Buckeye
Total Page:16
File Type:pdf, Size:1020Kb
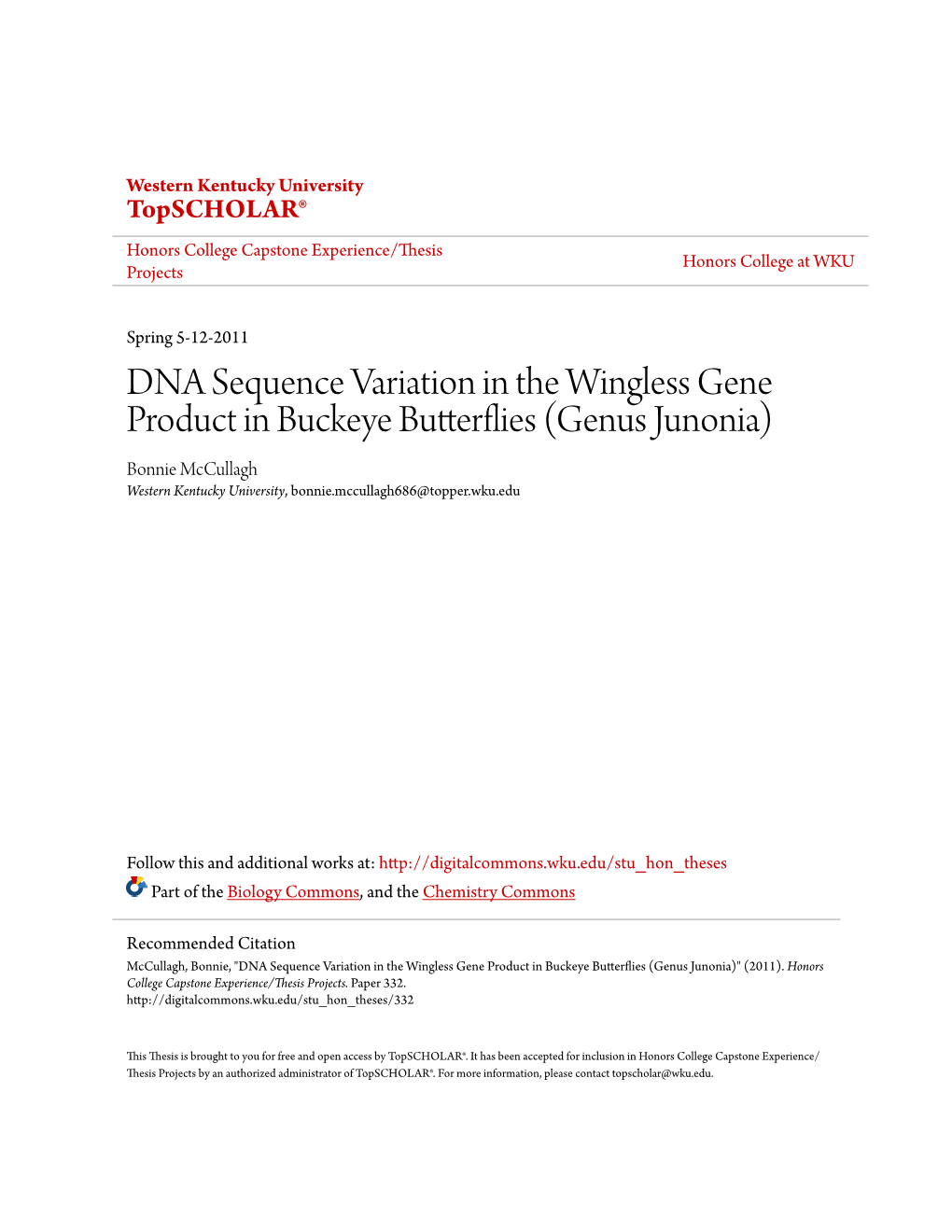
Load more
Recommended publications
-
A Molecular Phylogeny of the Neotropical Butterfly Genus Anartia
MOLECULAR PHYLOGENETICS AND EVOLUTION Molecular Phylogenetics and Evolution 26 (2003) 46–55 www.elsevier.com/locate/ympev A molecular phylogeny of the neotropical butterfly genus Anartia (Lepidoptera: Nymphalidae) Michael J. Blum,a,b,* Eldredge Bermingham,b and Kanchon Dasmahapatrab,c a Department of Biology, Duke University, Durham, NC 27705, USA b Smithsonian Tropical Research Institute, Naos Island Molecular Laboratories, Unit 0948, APO-AA 34002-0948, Panama, FL, USA c Department of Biology, Galton Laboratory, University College, 4 Stephenson Way, London NW1 2HE, UK Received 2 August 2001; received in revised form 17 June 2002 Abstract While Anartia butterflies have served as model organisms for research on the genetics of speciation, no phylogeny has been published to describe interspecific relationships. Here, we present a molecular phylogenetic analysis of Anartia species relationships, using both mitochondrial and nuclear genes. Analyses of both data sets confirm earlier predictions of sister species pairings based primarily on genital morphology. Yet both the mitochondrial and nuclear gene phylogenies demonstrate that Anartia jatrophae is not sister to all other Anartia species, but rather that it is sister to the Anartia fatima–Anartia amathea lineage. Traditional bi- ogeographic explanations for speciation across the genus relied on A. jatrophae being sister to its congeners. These explanations invoked allopatric divergence of sister species pairs and multiple sympatric speciation events to explain why A. jatrophae flies alongside all its congeners. The molecular phylogenies are more consistent with lineage divergence due to vicariance, and range expansion of A. jatrophae to explain its sympatry with congeners. Further interpretations of the tree topologies also suggest how morphological evolution and eco-geographic adaptation may have set species range boundaries. -
Diversidad Y Taxonomía De Mariposas Diurnas Del Macizo Acahay, Paraguarí, Paraguay
FICHA DE INSCRIPCIÓN 1) Nombre COMPLETO del autor1: Selma Suzanne Araceli Van Ruymbeke Ramos 2) Número de DNI: 3815882 3) Fecha de Nacimiento: 14/07/93 4) Pasaporte: N428119 5) Teléfono: 021-584396 6) Celular: +595 981 890519 7) Correo electrónico: 8) Estudiante de grado ….. Estudiante de posgrado….. Graduado…X. 9) Carrera: Lic. En Ciencias Mención Biología 10) Unidad académica de pertenencia: Facultad de Ciencias Exactas y Naturales 11) Tiene necesidades especiales? No 12) Nombre del director del proyecto: Christian Vogt 13) Correo electrónico del director: [email protected] 14) Teléfono del director: +595 985 783730 15) Otros autores del trabajo: Ezequiel Núñez Bustos 16) Título del trabajo: Diversidad y Taxonomía de mariposas diurnas del Macizo Acahay, Paraguarí, Paraguay 1 En caso de que el trabajo sea seleccionado, SOLAMENTE el autor consignado en este campo participará de las XXVI JJI de AUGM en la Universidad Nacional de Cuyo, Mendoza, Argentina. 17) Línea de investigación de AUGM a la que pertenece (marque con una cruz) A. Humanidades 1. A cien años de la Reforma Universitaria: saber te hace libre 2. Evaluación Institucional, Planeamiento Estratégico y Gestión Universitaria 3. Ciencias Políticas y Sociales 4. Desarrollo Regional 5. Educación para la Integración 6. Enseñanza de Español y Portugués como Lengua Segunda o Extranjera 7. Extensión Universitaria 8. Género 9. Historia, Regiones, y Fronteras 10. Literatura, Imaginarios, Estética, y Cultura 11. Medios y Comunicación Universitaria 12. Procesos Cooperativos y Asociativos 13. Producción Artística y Cultural 14. Accesibilidad y Discapacidad B. Ciencias Exactas 15. Biofísica 16. Ciencias e Ingeniería de Materiales 17. Ciencia, Tecnología, e innovación 18. -
A Review of Factors Associated with Decline and Death of Mangroves, with Particular Reference to Fungal Pathogens
SAJB-01189; No of Pages 7 South African Journal of Botany xxx (2014) xxx–xxx Contents lists available at ScienceDirect South African Journal of Botany journal homepage: www.elsevier.com/locate/sajb Review A review of factors associated with decline and death of mangroves, with particular reference to fungal pathogens J. Alexander Osorio a,1, Michael J. Wingfield a,1, Jolanda Roux b,⁎ a Department of Microbiology and Plant Pathology, DST/NRF Centre of Excellence, Forestry and Agricultural Biotechnology Institute (FABI), University of Pretoria, Private Bag X20, Hatfield, Pretoria 0028, South Africa b Department of Microbiology and Plant Pathology, DST/NRF Centre of Excellence in Tree Health Biotechnology (CTHB), Forestry and Agricultural Biotechnology Institute (FABI), University of Pretoria, Private Bag X20, Hatfield, Pretoria 0028, South Africa article info abstract Article history: Mangrove species grow in bays and estuaries in tropical and subtropical latitudes. Mangrove systems are catego- Received 1 July 2014 rized as highly productive, providing crucial environmental functions. Their stability and survival are, however, Received in revised form 19 August 2014 constantly threatened by anthropogenic activities and there has been an increase in reports of decline and Accepted 27 August 2014 death of these trees globally. Currently, little is known regarding diseases affecting mangroves, particularly Available online xxxx those caused by micro-organisms such as fungi. In recent years several studies of the fungi associated with fi Edited by J Van Staden these trees have been conducted and a number of fungal diseases have been identi ed. However, few studies have been done in South Africa and little is known regarding the health status of mangroves in the country. -
BUTTERFLIES in Thewest Indies of the Caribbean
PO Box 9021, Wilmington, DE 19809, USA E-mail: [email protected]@focusonnature.com Phone: Toll-free in USA 1-888-721-3555 oror 302/529-1876302/529-1876 BUTTERFLIES and MOTHS in the West Indies of the Caribbean in Antigua and Barbuda the Bahamas Barbados the Cayman Islands Cuba Dominica the Dominican Republic Guadeloupe Jamaica Montserrat Puerto Rico Saint Lucia Saint Vincent the Virgin Islands and the ABC islands of Aruba, Bonaire, and Curacao Butterflies in the Caribbean exclusively in Trinidad & Tobago are not in this list. Focus On Nature Tours in the Caribbean have been in: January, February, March, April, May, July, and December. Upper right photo: a HISPANIOLAN KING, Anetia jaegeri, photographed during the FONT tour in the Dominican Republic in February 2012. The genus is nearly entirely in West Indian islands, the species is nearly restricted to Hispaniola. This list of Butterflies of the West Indies compiled by Armas Hill Among the butterfly groupings in this list, links to: Swallowtails: family PAPILIONIDAE with the genera: Battus, Papilio, Parides Whites, Yellows, Sulphurs: family PIERIDAE Mimic-whites: subfamily DISMORPHIINAE with the genus: Dismorphia Subfamily PIERINAE withwith thethe genera:genera: Ascia,Ascia, Ganyra,Ganyra, Glutophrissa,Glutophrissa, MeleteMelete Subfamily COLIADINAE with the genera: Abaeis, Anteos, Aphrissa, Eurema, Kricogonia, Nathalis, Phoebis, Pyrisitia, Zerene Gossamer Wings: family LYCAENIDAE Hairstreaks: subfamily THECLINAE with the genera: Allosmaitia, Calycopis, Chlorostrymon, Cyanophrys, -
De Val De Serra, Região Central Do Rio Grande Do Sul, Brasil
Biota Neotrop., vol. 12, no. 2 Borboletas (Lepidoptera: Hesperioidea e Papilionoidea) de Val de Serra, região central do Rio Grande do Sul, Brasil Ana Beatriz Barros de Morais1,3, Renata Lemes1 & Camila Duarte Ritter2 1PPG Biodiversidade Animal, Centro de Ciências Naturais e Exatas, Universidade Federal de Santa Maria – UFSM, Faixa de Camobi, Km 09, CEP 97105-900, Santa Maria, RS, Brasil 2PPG em Ecologia, Instituto Nacional de Pesquisas na Amazônia – INPA, Av. André Araújo, 2936, Aleixo, CEP 69060-001, Manaus, AM, Brasil 3Autor para correspondência: Ana Beatriz Barros de Morais, e-mail: [email protected] MORAIS, A.B.B., LEMES, R. & RITTER, C.D. Butterflies (Lepidoptera: Papilionoidea) from Val de Serra, central region of Rio Grande do Sul State, Brazil. Biota Neotrop. 12(2): http://www.biotaneotropica.org.br/ v12n2/en/abstract?inventory+bn01412022012 Abstract: The butterfly fauna of the Atlantic Forest Biome is reasonably well-known up to the southern limit of its distribution. However, there are knowledge gaps nearby the central region of Rio Grande do Sul State, whose forest areas are considered priorities for biological conservation. This study investigated the butterfly assemblage of a riparian fragment in an ecotone area between Mixed Ombrophilous Forest and Seasonal Decidous Forest, in Itaara municipality. From September 2005 to September 2006, after 105 net-hours through active search sampling, 877 butterflies were registered representing 104 species. Three more species were registered posteriorly, increasing total richness to 107. The most abundant species were the Nymphalidae Hermeuptychia hermes (Fabricius, 1775), Yphthimoides celmis (Godart, [1824]), Agraulis vanillae maculosa (Stichel, [1908]), Tegosa claudina (Eschscholtz, 1821) and Vanessa braziliensis (Moore, 1883). -
Checklist of Butterflies (Insecta: Lepidoptera) from Serra Do Intendente State Park - Minas Gerais, Brazil
Biodiversity Data Journal 2: e3999 doi: 10.3897/BDJ.2.e3999 Taxonomic paper Checklist of butterflies (Insecta: Lepidoptera) from Serra do Intendente State Park - Minas Gerais, Brazil Izabella Nery†, Natalia Carvalho†, Henrique Paprocki† † Pontifícia Universidade Católica de Minas Gerais, Belo Horizonte, Brazil Corresponding author: Henrique Paprocki ([email protected]) Academic editor: Bong-Kyu Byun Received: 28 Aug 2014 | Accepted: 10 Nov 2014 | Published: 25 Nov 2014 Citation: Nery I, Carvalho N, Paprocki H (2014) Checklist of butterflies (Insecta: Lepidoptera) from Serra do Intendente State Park - Minas Gerais, Brazil. Biodiversity Data Journal 2: e3999. doi: 10.3897/BDJ.2.e3999 Abstract In order to contribute to the butterflies’ biodiversity knowledge at Serra do Intendente State Park - Minas Gerais, a study based on collections using Van Someren-Rydon traps and active search was performed. In this study, a total of 395 butterflies were collected, of which 327 were identified to species or morphospecies. 263 specimens were collected by the traps and 64 were collected using entomological hand-nets; 43 genera and 60 species were collected and identified. Keywords Espinhaço Mountain Range, Arthropoda, frugivorous butterflies, Peixe Tolo, inventory Introduction The Lepidoptera is comprised of butterflies and moths; it is one of the main orders of insects which has approximately 157,424 described species (Freitas and Marini-Filho 2011, Zhang 2011). The butterflies, object of this study, have approximately 19,000 species described worldwide (Heppner 1991). The occurrence of 3,300 species is estimated for © Nery I et al. This is an open access article distributed under the terms of the Creative Commons Attribution License (CC BY 4.0), which permits unrestricted use, distribution, and reproduction in any medium, provided the original author and source are credited. -
Journal of the Lepidopterists' Society
VOLUME 66, N UMBER 4 185 J OURNAL OF T HE L EPIDOPTERISTS ’ S OCIETY Volume 66 2012 Number 4 Journal of the Lepidopterists’ Society 66(4), 2012, 185 –198 DNA BARCODES AND INSIGHTS INTO THE RELATIONSHIPS AND SYSTEMATICS OF BUCKEYE BUTTERFLIES (NYMPHALIDAE: NYMPHALINAE: JUNONIA ) FROM THE AMERICAS EDWARD PFEILER , Centro de Investigación en Alimentación y Desarrollo, A.C., Unidad Guaymas, Apartado Postal 284, Guaymas, Sonora C.P. 85480, México; email: [email protected] SARAH JOHNSON , Division of Biological Sciences, University of California, San Diego, La Jolla, California 92093 AND THERESE A. M ARKOW Division of Biological Sciences, University of California, San Diego, La Jolla, California 92093 ABSTRACT. Nucleotide sequence data from a segment of the mitochondrial cytochrome c oxidase subunit I (COI) gene, known as the barcode segment, were used to examine phylogenetic relationships and systematics of buckeye butterflies (Nymphalidae: Nymphalinae: Junonia ) from the New World, with emphasis on taxa from western North America . Three nominal species have been recognized for North America, J. evarete (Cramer), J. genoveva (Cramer), and J. coenia Hübner, with additional species recently pro - posed for the West Indies and northern South America . The distinctive Andean buckeye, J. vestina C. Felder & R. Felder, along with J. evarete and J. genoveva , are also components of the South American fauna . With the exception of J. vestina , butterflies comprising the New World Junonia have had a confused taxonomic history, and species assignments are often problematic . Our results show that the barcode segment resolves the two major clades of New World Junonia , referred to here as clades A and B, with similar high sup - port seen in an earlier phylogenetic study using both mitochondrial and nuclear genes . -
Land Stewardship Plan Development and Supplemental Information Section That Can Be Found At: Ii
i Acknowledgements This document was prepared with the assistance of Lee County Conservation Lands Coordinators, Lee County Conservation 20/20 staff, Lee County Parks and Recreation staff and the Friends of Matanzas Pass Preserve. Many Thanks for your Time and Talent, Terry Cain & Sherry Furnari ii Table of Contents VISION STATEMENT ........................................................................................... 1 I. EXECUTIVE SUMMARY .................................................................................. 2 II. INTRODUCTION ............................................................................................. 3 III. LOCATION AND SITE DESCRIPTION .......................................................... 5 IV. NATURAL RESOURCES DESCRIPTION ..................................................... 8 A. Physical Resources ..................................................................................... 8 i. Climate ....................................................................................................... 8 ii. Geology ..................................................................................................... 8 iii. Topography .............................................................................................. 8 iv. Soils ....................................................................................................... 10 v. Hydrologic Components and Watershed ............................................... .12 B. Biological Resources ............................................................................... -
New Records of Pollinators and Other Insects Associated with Arizona Milkweed, Asclepias Angustifolia, at Four Sites in Southeastern Arizona
Journal of Pollination Ecology, 27(1), 2021, pp 1-24 NEW RECORDS OF POLLINATORS AND OTHER INSECTS ASSOCIATED WITH ARIZONA MILKWEED, ASCLEPIAS ANGUSTIFOLIA, AT FOUR SITES IN SOUTHEASTERN ARIZONA Robert A. Behrstock Naturewide Images, 10359 S Thicket Place, Hereford, AZ 85615 U.S.A. Abstract—Asclepias angustifolia is a Mexican milkweed that barely enters the U.S.A. Its pollinators and other insect visitors have not been investigated. During 2018 and 2019, insect visitors were photographed at a native population and three gardens in and near the Huachuca Mountains, Southeastern Arizona. A total of 216 site visits produced at least 369 species of insects in seven orders. Images revealed 140 potential pollinators with a preponderance of Hymenoptera, Lepidoptera, and Diptera. Orders of insects are discussed, as are flowering phenology, potential pollinators in functional groups, introduced insects, and the value of A. angustifolia for monarch butterflies and other insects in pollinator gardens and in planting palettes created for restoration sites. Keywords: Sky Island, Madrean Pine-Oak Woodland, monarch butterfly, Huachuca Mountains, gardening, restoration INTRODUCTION milkweed, A. linaria Cavanillies, that produces higher concentrations of cardenolide toxins and greater amounts of North American milkweeds (Asclepias spp.) provide defensive latex (Pegram & Melkonoff 2019). Planting nectar to an unusually large diversity of insects, making them milkweeds is becoming a widespread practice aimed at important members of existing ecosystems and valuable increasing north- or southbound cohorts of the monarch’s additions to sites benefiting from a broad spectrum of complicated multi-generational migration; however, some pollinators (Ollerton et al. 2019, Tallamy 2007). For authors (e.g., Inamine et al. -
Big Cypress Butterflies Harmonize with the Plants of the Swamp by Distributing Pollen on Their Bodies As They Fly from Plant to Plant in Search of Nectar
National Park Service U.S. Department of the Interior Big Cypress National Preserve Florida Butterflies of the Swamp... The word “Butterfly” translated in most languages showcases beautiful rhythimic words that echo Big Cypress the beauty of the insect and its repetitive wing beats. For example, in Spanish: Mar-i-po’-sa, French: Papillon, German: Shmetterling, and Tamil: Butterflies Vannathi poochi to name a few. In Big Cypress butterflies harmonize with the plants of the swamp by distributing pollen on their bodies as they fly from plant to plant in search of nectar. Recommended Butterfly Sites Within the Preserve check out the Fire Prairie Trail Watching wildlife the off of Turner River Road, Gator Hook Trail, Florida responsible way... National Scenic Trail. Or take a stroll through The thrill of watching a wild animal in the Oasis Visitor Center native plant garden and observe butterflies fluttering from plant to plant. its native surroundings is spectacular and awe inspiring. While visiting Big The best time to see butterflies in the Preserve is Cypress National Preserve, or any other in the late summer and autumn months from late natural area, remember: August to mid-October. At this time there is a wide variety and abundance of butterflies. The winter months from November through February are • All wildlife is wild and unpredictable. more limited. In early spring the first generation Stay a safe distance from any wild of Gray Hairstreaks and swallowtails are first animal —15 feet is recommended. to appear. In late spring into summer butterfly • View wildlife with respect. numbers increase with new generatons. -
Field Guide to the Butterflies of the Springfield Plantation
Field Guide to the butterflies of the Springfield Plantation Tasha Bedgood Texas A&M University Study Abroad Program Dr. Tom Lacher Dr. Robert Wharton Dominica 2001 Tasha Bedgood Dominica 2001 Field Guide to the butterflies of the Springfield Plantation Abstract Butterflies are in the order Lepidoptera and I have found six different families and sixteen different species. The butterflies that were found on the trail to the right of the veranda consisted of a variety of different species, as opposed to some of the other locations that contained only a few different species. The Caribbean Buckeye was the one species of butterfly that seemed to be grouped in one area. They are found in great quantities at Mt. Joy. Introduction Dominica is a beautiful island with many natural attractions and historical sites. One of the attractions on the island is the Springfield Plantation. It is an old plantation that has not been in operation for some time. Clemson University bought the plantation in 1989 and renamed it The Archbold Tropical Research Center. The plantation is now used as a guesthouse and a center for environmental protection, research, and education. Springfield is in a transitional or deciduous forest, which allows researchers to study a variety of species of the flora and fauna. While I was at the center, I chose to study the butterflies on the plantation. Butterflies are beautiful creatures that catch the eye of anyone they encounter. I did a survey of the butterflies and recorded the species in different areas. Method and Materials I caught and identified a number of butterflies around the field station (veranda), the stream house, down a path to the right of the veranda, at the Bee House, and at Mt. -
Garden Fitness Jeopardy
Mangrove Conservation Jeopardy Thomas Becker, FFL Program Assistant Charlotte County Extension Service 10-20-15 What’s Missing in this picture Goal: Learn innumerable benefits of mangrove and proper maintenance Objectives • Demonstrate ‘How to’ apply a ppt./2010 jeopardy board template to a presentation • Adopt accepted norms relating to mangrove ecology, preservation, restoration and care • Understand the role and values of mangroves • Learn how to identify the differences between three mangrove species and those plants that often grow nearby Mangrove – red, black & white Constructed Canal Lot Forest, Eco- System, Fringe, Buffer, Back-bay Border, Swamps Wildlife Corridors, Tunnels, Remnants, Tree Islands, Aquatic Estuary Natural Mangrove along Gasparilla Island World Mangrove Distribution *National Geographic Magazine Charlotte Harbor Myakka River Peace River Pt Charlotte Lemon Bay Punta Gorda 18 Miles To Caloosahatchee Boca Grande Gulf River Cape Coral Captiva Ft Myers Sanibel Estero Bay What is an Estuary? Where fresh water meets salt water supporting GREAT plant and wildlife bio-diversity. Kidneys for the watershed improving water quality before going into the Gulf of Mexico. Charlotte Harbor estuary Shallow Water High biological diversity • Rivers • Barrier islands • Open water • Inlets • Salt marshes • Submerged • Oyster bars • Mud flats seagrass beds Species Living in SWFL Estuary Osprey Inshore lizardfish • 227 species of birds • 225 species of fish • 22 native species of • 468 native species of plants mammals including 84 non-natives